Sponsored by HORIBAJun 12 2018
Diffusion is a process that has been known and used for centuries. In the ancient times, people who dyed fabrics began to notice the cause of spreading turquoise dye in their cotton cloth; biologists, on the other hand, have discovered the process of osmosis in various particles. With advanced engineered materials propagating throughout the 21st century, scientists and engineers now have the capability of designing diffusive layers in order to provide controlled rates of selective diffusion.
Diffusion in Current Technology
A number of applications in engineered diffusion exist in current technology. Some of these advances are applied medically, such as drug patches that allow the sustained slow release of pharmaceuticals or membranes that are used in dialysis and other laboratory procedures. Similar to the use of drug patches, the agricultural application of engineered diffusion could be attributed to slow release nodules that provide sustained fertilization.
Meanwhile, the industrial production of chemicals and food products often depend on separations with various membranes. Other pertinent applications of engineered diffusion are within less organic natures, including the separation of gases by selective diffusion or the segregation of ions through a solid (such as a part of a battery or electronic device). At times, diffusion may be undesirable. This may occur when gases dissolved in a solid need to be stored or when the diffusion of oxygen into a semiconductor crystal can depreciate the performance of an electronic device.
In order to develop materials that have controlled diffusive properties, the diffusive process needs to be measured in situ. In order to measure diffusion in a microscopic scale, a limited number of analytical tools are available for use. An ideal diffusion technique would be one that (1) provides molecular information with resolution on a microscopic scale, (2) is non-destructive of the samples, and (3) could be set up and used on a laboratory bench top with minimal time and effort in preparation.
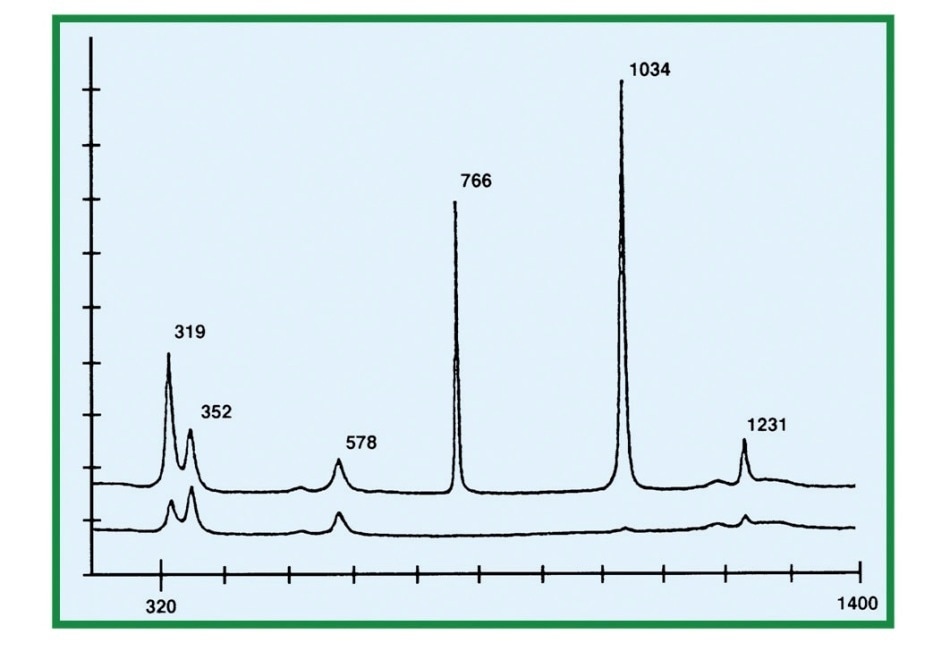
Figure 1. Spectrum of Triflate in Water
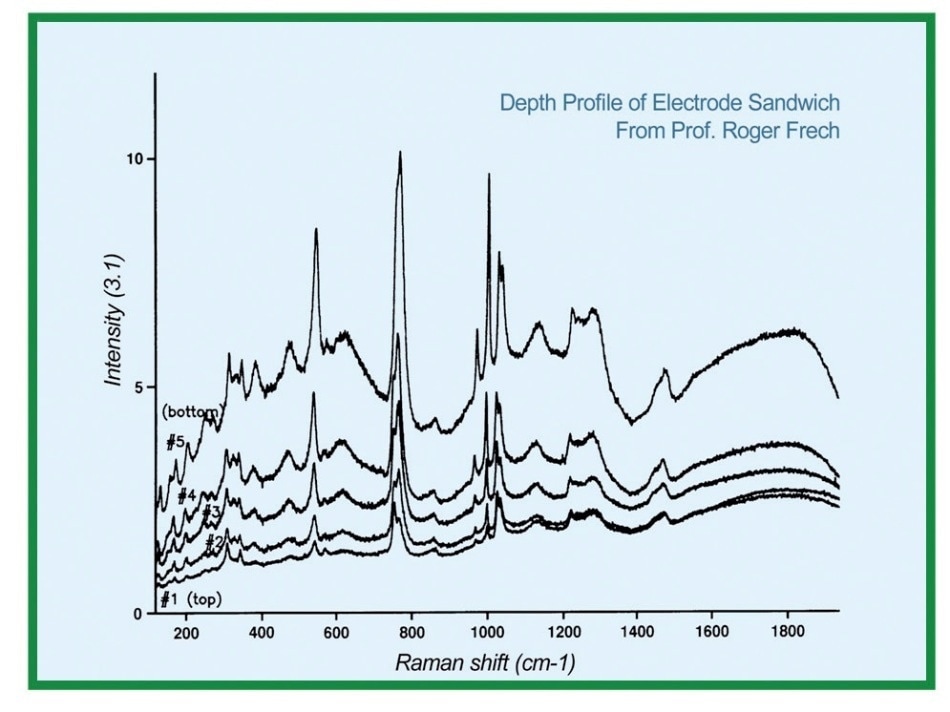
Figure 2. Spectra taken at different depths within the polymer layer
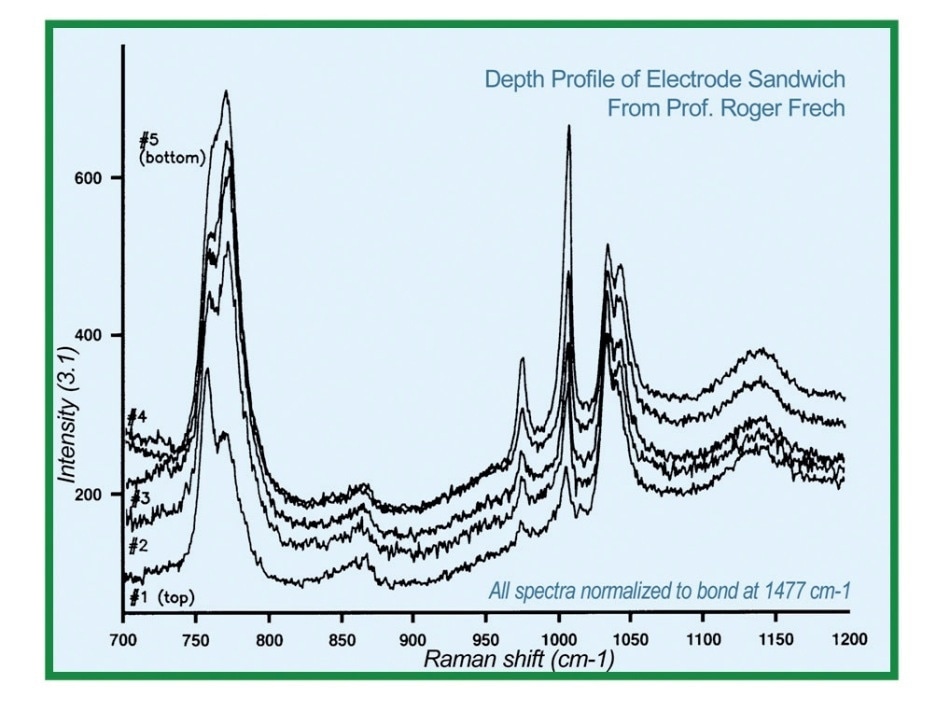
Figure 3. Spectra taken at different depths within the polymer layerwith normalized spectra
Raman Microprobe Spectroscopy
An ideal candidate in meeting such needs is Raman microprobe spectroscopy. The use of visible light coupled with a confocal microscope enables a probe with spatial resolution of a micron at the minimum. The coupling of such microscope to a modern Raman spectrometer equipped with holographic notch filters and CCD multi-channel detectors would allow the rapid acquisition of Raman spectra which could then be correlated with the chemical state of the species and its physical environment.
In the 1950s, the confocal microscope was invented. Decades later, it has become a popular choice in scientific applications. The efficient performance of confocal microscopy is proven by various published articles, notably the Scientific American of August 1994 and Photonics Spectra of February 1995. The Raman microprobe that utilizes the injection of a laser beam into a confocal microscope, coupled with the spatially resolved output of the confocal microscope to a Raman spectrometer was a mechanism developed in the early 1980s but has now become a key player in obtaining information from various types of rather unobtainable samples, on a scale of micrometers. In contrast, infrared microscopes would only have resolutions between 5 to 10 micrometers at best.
This article aims to demonstrate the utility of the Raman microprobe by discussing a single set of measurements that was conducted on a model for a micro-battery diffusion layer.
Example: Diffusion in Microbatteries
Microbatteries are a salient part of miniaturizing all types of electronic devices. Batteries used in portable electronic devices need to be compact and light enough to be considered portable. Currently, microbatteries that are utilized in popular laptop computers use ionic conductivity rather than electrons to conduct charge. Lithium is the typical ion of choice. The components of the battery must always allow movement of the lithium ions.
In the battery model probed in the demonstration, a layer of polyethylene oxide was cast on a vanadium oxide ceramic substrate, which served as the model electrode. The lithium ions conduct charge by diffusing from the electrode through the polymer layer. Being positively charged, the lithium ions need a static countering in the diffusion layer in order to provide electrical neutrality. In this process, the trifluoromethanesulfonate anion is used. The use of Raman spectrum of trifluoromethanesulfonic acid dissolved in water yielded several sharp bands, particularly, sharp single bands at 766 and 1034 cm-1.
About 20 micrometers thick, the polyethyleneoxide layer is transparent to visible light. As such, the excitation laser beam could easily be focused at different depths within the samples by simply changing the height of the microscope stage.
A separate measurement of the index of refraction of the polyethyleneoxide medium would allowed the correction of the calibrated stage displacement to determine the exact depths of focus; nevertheless, the spectra continued to progress. When the raw spectra were corrected to account for the decrease in absolute intensity due to varied path length of both the laser and scattered radiation, significant results were expected to arise. This process was done by normalizing all spectra to one of the bands of the polymer matrix, specifically the one at 1477 cm-1.
After the process, two triflate bands which appeared as singlets in the aqueous spectra, at 766 and 1034 cm-1, were each split into two overlapped bands. Moreover, the relative proportions of the two components of the doublet at 766 cm-1 were altered with depth in the sample. The higher frequency component of the doublet increased in relative intensity as the bottom of the polymer layer and the interface with the ceramic electrode, was approached.
A crystal structure of such polymer electrolyte system was published by P. Lightfoot et al in 1993. Their research found that lithium ions are coordinated to five oxygen atoms, from either triflate ions or the host polymer. They also found that oxygen atoms of the triflate could either be coordinated (to lithium) or uncoordinated. The splitting of the triflate bands could therefore be generated from separate populations of coordinated and uncoordinated oxygen atoms, with the coordination shifting the Raman frequency.
The more lithium ions present in the system, the greater would be the expected population of triflate oxygen atoms with charged neighbors. In another study, this time focusing on homogeneous samples of triflate ion in the presence of various concentrations of lithium, an analysis of the doublet progression with either curve-fitting or chemometrics software was sought.
The result of such study would provide specific information regarding the chemical environment of the triflate ion, as well as the lithium concentration as functions of the depth. These measurements were performed with a sample with zero applied voltage. Further studies focused on spectra at fixed depth as a function of applied voltage or time of the applied voltage.
Measurement of Diffusion Processes
Similarly, the spatial and temporal dependencies of various other diffusion processes through transparent media may be quantified. In cases in which the sample can be sliced or microtomed, the automated mapping stage driven by SpectraMax software could allow the automatic collection of spectra as a function of depth.
This is enabled by providing a Raman map of spectra. This method provides an application of the use of Raman microscopy in obtaining useful and otherwise unobtainable information about a sample with a concentration gradient resulting from a diffusion process.

This information has been sourced, reviewed and adapted from materials provided by HORIBA.
For more information on this source, please visit HORIBA.