The following article is the second of a three-part series on a novel nanoelectrical imaging technique that goes beyond a 2D map, and produces a correlated nanomechanical DataCube and comprehensive electrical DataCube.
Case Study: DataCube Tunneling AFM (DCUBE-TUNA) on Li-Ion Battery Cathodes
Complex interrelated phenomena lead to battery failure, which is dependent on battery design, environment, chemistry, and true operation conditions. Therefore, studies at the component level are required for inferring, enhancing, and regulating the performance and lifetime of a battery. AFM has been shown to be a robust tool for battery component research. Yet, the presence of materials with clear mechanical characteristics, from loose (conductive additive), soft (polymer additive), to porous (separator), hard (Li metal oxide) surfaces, and high difference in surface topography, can pose difficulties for contact-based scanning modes owing to the nature of high normal shear force and imaging force.
In this regard, tapping mode is better than contact mode surface mapping, but most of the nanoelectrical characterization is predicated on the contact between the sample and the solid tip. In the recent past, PeakForce Tapping modes have been shown to be handy for imaging complex samples like battery materials, specifically for in-situ analyses of the delicate solid electrolyte interphase layer present on anodes and cathodes. The capabilities of PeakForce Tapping modes are further expanded by DCUBE modes by allowing the acquisition of multidimensional data cubes through the concurrent capture of nanometer-scale mechanical and electrical properties in high-density data cubes.
In this case, the cathode investigated contains conductive carbon nanoparticles, polymer binder, and Li metal oxide. Firstly, a large 45 x 45 μm2 area was scanned, which can be difficult for other AFM nanoelectrical techniques on this kind of composite sample. A high-quality image of surface topography is illustrated in Figure 5a. The height variation is more than 1 μm, which is considered normal for a Li-ion battery cathode. Within the images, representative domains of Li metal oxide (1), polymer binder (2), and carbon black (3) are shown.
.jpg)
Figure 5. DCUBE-TUNA study of a battery cathode consisting of Li metal oxide (1), polymer binder (2), and conductive carbon nanoparticles (3): (a) surface topography, (b) quantitative surface stiffness differentiating different domains, and (c) a collection of TUNA current slices from the spectroscopic mapping at selected sample voltages. The scanning area is 45 x 45 μm2.
Domains like these are better recognized by Figure 5b, a quantitative map of surface stiffness, since the polymer binder is the softest material and shows the lowest stiffness, while Li metal oxide is the hardest material and displays the highest stiffness. A series of slices extracted from the spectroscopic mapping is shown in Figure 5c, demonstrating the TUNA current image at the chosen sample bias, as specified to the right. The TUNA current maps reveal not just clear conductivities over varied material domains but also conductivity heterogeneity over varied Li metal oxide grains.
Additional data about this sample with a scan size of 15 x 15 μm2 are shown in Figure 6, where the varied material domains, as well as the heterogeneity over varied Li metal oxide grains, are additionally indicated. The surface topography in Figure 6a reveals a few large Li metal oxide grains, for example, regions #1 to #3. It is hard to detect the inter-grain filling materials on this morphology map. This is partly dealt with the stiffness map and the modulus map shown in Figure 6b and 6c, where high stiffness and modulus are respectively exhibited by Li metal oxide.
Such a differentiation is validated by the TUNA current map shown in Figure 6d — a slice of spectroscopic mapping at +4 V sample bias. High conductivity is exhibited by carbon black-rich regions (region #5), while the polymer binder regions are observed to be insulating (region #4). It is interesting to note that grain #2 for the Li metal oxide domains displays only background current, which is completely different from other types of grains. This may be attributed to the absence of conduction channel linked by the inherent nature of the grain itself or by carbon black additives.
It is essential to avoid this region in practical design since it only acts as a redundant volume and weight burden to the battery, decreasing the particular energy or volumetric energy density. Furthermore, the Li metal oxide exhibits inner-grain heterogeneity, as specified by grain #3 in Figure 6d. Figure 6e shows a series of slices from the spectroscopic mapping, indicating TUNA current images at designated sample biases as indicated. The electrical characteristics are further confirmed by this collection of images. More significantly, it demonstrates the dynamic variations with applied sample biases from −4 V to +4 V. Within the Li metal oxide grains, the current-voltage behaviors appear to deviate from linear Ohmic response; for instance, the cathode is comparatively silent to sample bias from −0.5 V to +0.25 V.
.jpg)
Figure 6. DCUBE-TUNA study of a battery cathode consisting of Li metal oxide (1)–(3), polymer binder (4), and conductive carbon nanoparticles (5): (a) surface topography, (b) quantitative surface stiffness differentiating different domains, (c) quantitative modulus map, and (d)–(e) TUNA current slices from the spectroscopic mapping at selected sample voltages. The scanning area is 15 x 15 μm2.
The DCUBE-TUNA results of one Li metal oxide grain on this cathode are shown in Figure 7. Figure 7a shows surface topography, indicating that this grain is an aggregate of sub-micron nanoparticles having analogous mechanical characteristics, as demonstrated in the stiffness and modulus in Figure 7b and Figure 7c, respectively.
.jpg)
Figure 7. DCUBE-TUNA studies of a Li metal oxide grain on a battery cathode consisting of Li metal oxide, polymer binder, and conductive carbon nanoparticles: (a) surface topography; (b) map of quantitative surface stiffness differentiating different domains; (c) quantitative modulus map; (d)–(e) two slices from spectroscopic mapping showing TUNA current image at a sample bias of +4 V and −4 V, respectively; and (f) all current-time spectra from the area indicated by the white square in (e). During this 125 ms dwell period, the sample bias sweeps linearly from −4 V to +4 V, or at a rate of 64 V·s−1.
In Figure 8d and 8e, the TUNA current slices obtained from the DCUBE-TUNA spectra at +4 V and −4 V sample biases demonstrate conductivity differences among these particles.
.jpg)
Figure 8. DCUBE-SCM study of two adjacent SRAM pnp transistors. This collection of images displays the SCM dC/dV amplitude data: (a) data cube in a 3D space of X, Y, and VDC; (b) a slice of the data cube at a fixed Y position; (c)–(k) slices at selected, fixed DC biases along the VDC coordinate. DC sample voltage was swept from −2 V to +2 V. The scan size is 2 x 2 μm2 with 128 x 128 pixels.
This proves the inner-grain electrical heterogeneity illustrated in Figure 6d. All current-time spectra from the area denoted by the white square in Figure 7e are plotted in Figure 7f. During this dwell time of 125 ms, the sample bias linearly sweeps from −4 V to +4 V, or at a speed of 64 V/second. The square area is across a pair of nanoparticles with varied conductivities, as illustrated in Figure 7e. The conductivity of each particle is comparatively uniform, resulting in two clear spectral groups, as illustrated in Figure 7e.
This DCUBE-TUNA example clearly shows the importance of dynamic, multidimensional data for distinctly distinguishing and examining the performance of an intricate system built from functional materials with clear physical characteristics.
Case Study: DataCube Scanning Capacitance Microscopy (DCUBE-SCM) of Semiconductor Devices
Through the SCM technique, activated carrier concentration can be directly measured with nanometer-scale precision in two dimensions. In this method, the difference in the carrier concentration within semiconductor structures can be imaged by measuring the dC/dV signal of the metal-oxide-semiconductor (MOS) capacitor created by the semiconductor sample and the probe. In this specific mode, the probe is scanned in contact mode and electrical and topography data are simultaneously obtained, making it possible to directly correlate the location of a sample with its electrical characteristics. SCM’s applications include 2D carrier profiling of cross-sectioned semiconductor devices and failure analysis of semiconductor devices.
Two limitations are faced in traditional SCM. Firstly, while the SCM works in contact mode, it can experience high imaging and shear forces, which are directly linked to the lifetime of the tip, particularly on structures that have considerable topography differences. Secondly, it works at a constant DC bias voltage between the sample and the tip, while plenty of data becomes apparent only when operating at a wide range of voltages. As a matter of fact, cycling DC bias on each pixel has been an interesting topic for quite some time.15 By contrast, DCUBE-SCM resolves both these limitations by offering high spectral and spatial resolution spectroscopic mapping data without using the contact mode. Through an additional examination of the ensuing data cubes, scientists can gain more information on threshold voltages, oxide charges, oxide thickness, interface trap densities, and contamination from mobile ions.
A DCUBE-SCM example from an SRAM transistor is shown in Figure 8. The DC sample voltage in this kind of measurement was swept from −2 V to 2 V, with the scan size being 2 x 2 μm2 with 128 x 128 pixels. Every pixel took 100 ms and the entire whole data cube was collected in 27 minutes. Apart from the force-distance data, both the SCM dC/dV amplitude (shown in Figure 8) and the dC/dV phase data were stored. As illustrated in Figure 8a, the visualization of the dC/dV amplitude data cube in a 3D space of X, Y, and VDC was generated in MATLAB. Figure 8b shows the vertical plane, which is a slice revealing the dynamic change of dC/dV amplitude with VDC along the line of the X direction.
This slice demonstrates the way the pnp junction carrier profile differs with the DC sample voltage and is an indication of the movement of “apparent junction,” in other words, effective channel length with DC voltage. Figure 8c to 8k shows slices obtained at fixed DC voltages, with each image indicating the fixed DC voltage. A defect on the right pnp junction is shown in Figure 8e and 8i.
Fascinatingly, this defect can only be seen at specific voltages, as demonstrated in this sequence of data. A movie of the entire collection of 128 slices can show this defect in a better way.
This clearly shows how DataCube imaging makes it possible to view effects that are easily missed when utilizing traditional electrical modes operated at just a few distinct operating conditions.
References
- Palacin, M. R. and de Guibert, A., “Why Do Batteries Fail?” Science 351, 1253292 (2016).
- Semenov, A. E., Borodina, I. N. and Garofalini, S. H., “In Situ Deposition and Ultrahigh Vacuum STM/AFM Study of V2O5/Li3PO4 Interface in a Rechargeable Lithium-Ion Battery,” J Electrochem Soc 148, A1239-46 (2001).
- Becker, C. R., Prokes, S. M. and Love, C. T., “Enhanced Lithiation Cycle Stability of ALD-Coated Confined a-Si Microstructures Determined Using In Situ AFM,” ACS Appl Mater Inter 8, 530-37 (2016).
- Hernandez, G. et al., “Redox-Active Polyimide-Polyether Block Copolymers as Electrode Materials for Lithium Batteries,” RSC Adv 5, 17096-103 (2015).
- Hiesgen, R. et al., “AFM As an Analysis Tool for High-Capacity Sulfur Cathodes for Li-S Batteries,” Beilstein J Nanotech 4, 611-24 (2013).
- Chen, J. C., Yan, Y. D., Sun, T., Qi, Y. and Li, X. D., “Deformation and Fracture Behaviors of Microporous Polymer Separators for Lithium Ion Batteries,” RSC Adv 4, 14904-14 (2014).
- Breitung, B., Baumann, P., Sommer, H., Janek, J. and Brezesinski, T., “In Situ and Operando Atomic Force Microscopy of High-Capacity Nano-Silicon Based Electrodes for Lithium-Ion Batteries,” Nanoscale 8, 14048- 56 (2016).
- Shen, C. et al., “Direct Observation of the Growth of Lithium Dendrites on Graphite Anodes by Operando EC-AFM,” Small Methods 2, 1700289 (2018).
- Wang, S. W. et al., “Operando Study of Fe3O4 Anodes by Electrochemical Atomic Force Microscopy,” Appl Surf Sci 426, 217-23 (2017).
- Kumar, R. et al., “In Situ and Operando Investigations of Failure Mechanisms of the Solid Electrolyte Interphase on Silicon Electrodes,” ACS Energy Lett 1, 689-97 (2016).
- Wang, S. W., Yang, K., Gao, F., Wang, D. Y. and Shen, C., “Direct Visualization of Solid Electrolyte Interphase on Li4Ti5O12 by In Situ AFM,” RSC Adv 6, 77105-10 (2016).
- Kitta, M. and Kohyama, M., “Stability of the LiMn2O4 Surface in a LiPF6-Based Non-Aqueous Electrolyte Studied by In-Situ Atomic Force Microscopy,” Jpn J Appl Phys 55 (2016).
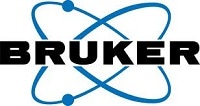
This information has been sourced, reviewed and adapted from materials provided by Bruker Nano Surfaces.
For more information on this source, please visit Bruker Nano Surfaces.