Organ-on-chip (OOC) devices combine advanced cell cultures with microengineering to create cutting-edge biotechnology promising to revolutionize sectors from drug development to chemical risk assessment.
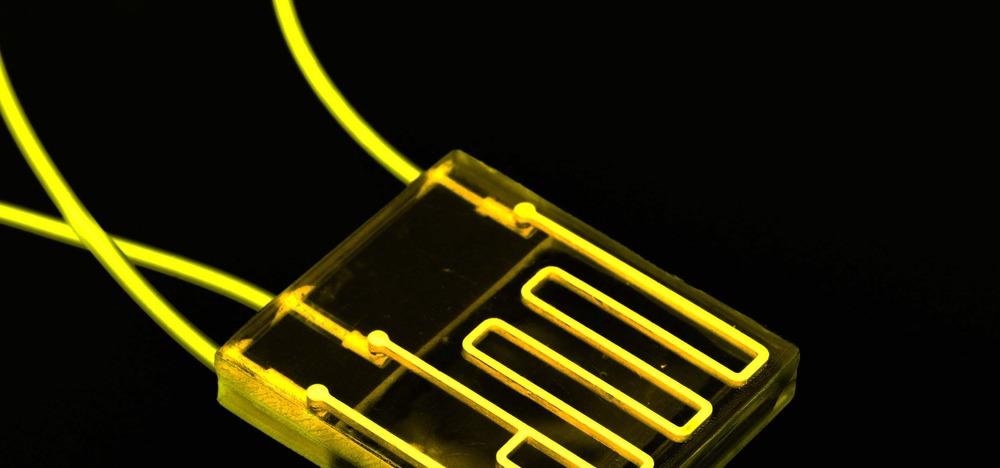
Image Credit: luchschenF/Shutterstock.com
What are Organ-on-Chip Devices?
An organ-on-chip (OOC) device is a micro-scale biomimetic system that replicates the complex structures and key functions of living organs. They do so by controlling the movement of fluids through miniature channels, membranes, and chambers lined with living human cells.
Their design differs depending on the organ in question but can be broadly categorized into single, double, and multi-channel chips. The most common is the double channel design, which has been used to study organs such as the lungs, intestine, and blood-brain barrier. Further design considerations, such as the chamber shape, channel diameter, and membrane properties, are also organ-specific and chosen accordingly.
The fluid flow rate within the channels is another organ-specific parameter that must be accurately and precisely controlled to set the desired fluid shear stresses, nutrient and oxygen concentration gradients, polarity, and various other critical parameters within the system.
This is often achieved through pressure-controlled flow with syringe pumps, but other approaches such as peristaltic, electro-osmotic, or capillary pumps are also used. Complex multichannel devices have also necessitated the use of microvalves to control fluid movement. These may be passive in nature, such as siphon or hydrophobic vales, or active, such as pneumatic or electrostatic valves.
The devices are similar in size to a USB memory stick with their micro-scale features typically created through fabrication methods such as soft lithography, hot embossing, and even 3D printing. Materials to make these devices must be optically transparent (to allow researchers to view the system), biocompatible (so as not to improperly affect the system) and cost-effective.
As such, silicon-based elastomer polydimethylsiloxane (PDMS) is commonly used for devices where flexibility is required, and glass for devices where it is not. Thermoplastics have also gained recent attention due to their chemical stability and suitability for efficient manufacturing methods such as injection molding and laser cutting.
History
The origins of in vitro research date back to the early twentieth century, when simple semi-controlled 2D culture techniques gave basic insights into cell growth and differentiation. Since then, techniques have been developed to provide the physiological and mechanical conditions experienced in the body (the in vivo conditions) with increasing accuracy.
This has led to 3D culture models, such as spheroid, organoid, and bioprinted tissue, which can accommodate the natural shape changes and cell-cell connections previously prohibited by the rigid 2D culture substrate.
OOC devices form the next progression of 3D culture models, offering enhanced dynamic functionality over traditional 3D cultures thanks to their use of micro-scale fluid flow, termed microfluidics. Although researched for over 20 years, the devices remain in their infancy with the first successful chip created only in 2010 by Harvard’s Wyss Institute.
Since then the technology has grown rapidly, with devices now able to successfully imitate a wide range of organs with incredible accuracy.
Applications
One of the main applications for OOC devices is in evaluating the safety of drugs prior to entering clinical trials. Current in vitro preclinical testing methods rely upon 2D and simplistic 3D culture models, which give often misleading results due to their inaccuracy in replicating the in vivo physiological conditions.
The same inaccuracy can be said for animal testing. This results in a notoriously slow and expensive drug discovery and development process – drug approval rates for clinical trials are approximately 12%, with each compound taking up to 10 years and $3 billion to bring to market (Wouters et al., 2020).
OOC devices are already displaying their advantages for this discovery and development purpose. For example, Kostrzewski et al., (2019) developed a liver-on-chip device that enabled unforeseen insights into the underlying mechanism behind the most common chronic liver disease in developed countries, non-alcoholic fatty liver disease (NAFLD). These insights were impossible to make using the traditional and inherently static 3D culture models, and are predicted to lead to the first successful pharmacological therapeutic options for the prevalent disease.
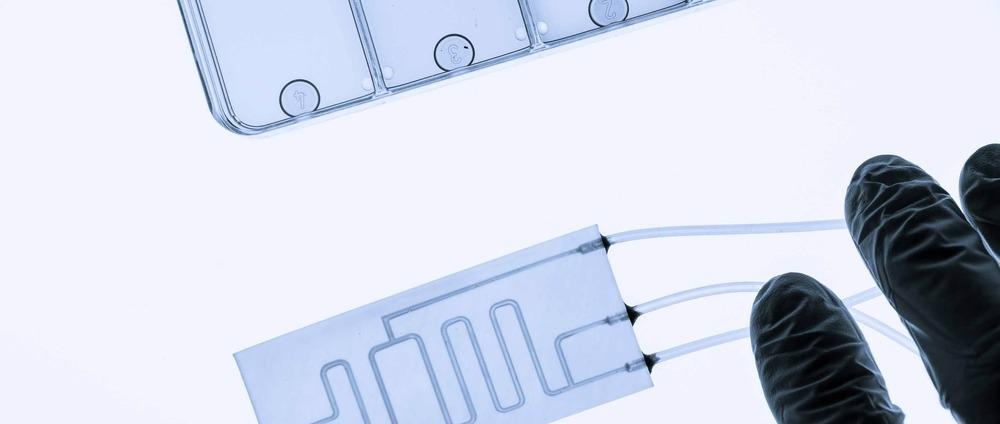
Image Credit: luchschenF/Shutterstock.com
Other OOC applications include lung assistance devices for preterm infants during respiratory failure, the development of highly personalized medicine, and the modeling of infectious diseases including COVID-19.
Challenges
Many crucial barriers exist before the widespread acceptance of OOC devices, particularly concerning their standardization and validation. This is because the devices not only comprise complex and interdisciplinary emerging technologies, currently making standardization extremely challenging and arguably counterproductive, but also neither animal models nor human clinical effects can be regarded as unequivocal standards with which to measure the validity of the devices. This makes the applicability of a validation process questionable, with some favoring the more focused steps of a qualification process.
A wide range of technical challenges form further barriers to device acceptance. This includes the unselective absorption of hydrophobic drugs, especially for PDMS-based OOC devices. This limitation is a result of the material’s tendency to mix with an oily phase (termed its lipophilicity) which restricts the possible drug delivery methods for the device and can drastically hinder the interpretation of its analytical results (Tajeddin and Mustafaoglu, 2021).
Other technical challenges include the integration of sensors to enable real-time system monitoring and automation, the limited scalability of device manufacturing techniques, the efficient isolation of quality primary cells to use for tissue sourcing within the devices, and the detrimental consequences of the dominating surface effects for micro-scale fluid flow.
Future Developments
The underlying trend within the technology, and arguably its ultimate goal, is to integrate more and more organs on a single chip to finally create a complete ‘body-on-chip’. Such a device would consist of multiple suitably scaled and functionally connected organs to give a testing environment of unprecedented completeness and accuracy.
In turn, this ‘multiplexing’ trend will undoubtedly present new and demanding challenges requiring a variety of further research and development. One such emergent development is the incorporation of stem cells as a tissue source for devices.
These ‘self-renewing’ cells that can differentiate into one or more specialized cell types are more physiologically representative than other cell lines and are predicted to become the main tissue source for future OOC devices (Wu et al., 2020). Other examples include the use of functional hydrogels, the development of vascularized OOC devices, and the implementation of automated closed-loop system control (Tajeddin and Mustafaoglu, 2021; Aazmi et al., 2021).
References and Further Reading
Aazmi, A., Zhou, H., Li, Y., Yu, M., Xu, X., Wu, Y., Ma, L., Zhang, B. and Yang, H., 2021. Engineered Vasculature for Organ-on-a-Chip Systems. Engineering. https://www.sciencedirect.com/science/article/pii/S2095809921003337
Ingber, D., 2022. Human Organs-on-Chips. [online] Wyss Institute. Available at: <https://wyss.harvard.edu/technology/human-organs-on-chips/#:~:text=4%2F7%20The%20Organs%2Don,that%20mimic%20organ%2Dlevel%20physiology>
Kostrzewski, T., Maraver, P., Ouro‐Gnao, L., Levi, A., Snow, S., Miedzik, A., Rombouts, K. and Hughes, D., 2019. A Microphysiological System for Studying Nonalcoholic Steatohepatitis. Hepatology Communications, 4(1), pp.77-91. https://aasldpubs.onlinelibrary.wiley.com/doi/full/10.1002/hep4.1450
Peng, J., Rochow, N., Dabaghi, M., Bozanovic, R., Jansen, J., Predescu, D., DeFrance, B., Lee, S., Fusch, G., Ravi Selvaganapathy, P. and Fusch, C., 2018. Postnatal dilatation of umbilical cord vessels and its impact on wall integrity: Prerequisite for the artificial placenta. The International Journal of Artificial Organs, 41(7), pp.393-399. https://pubmed.ncbi.nlm.nih.gov/29562805/
Tajeddin, A. and Mustafaoglu, N., 2021. Design and Fabrication of Organ-on-Chips: Promises and Challenges. Micromachines, 12(12), p.1443. https://www.mdpi.com/2072-666X/12/12/1443
Van den Berg, A., Mummery, C., Passier, R. and van der Meer, A., 2019. Personalised organs-on-chips: functional testing for precision medicine. Lab on a Chip, 19(2), pp.198-205. https://pubs.rsc.org/en/content/articlelanding/2019/lc/c8lc00827b
Wang, Y., Wang, P. and Qin, J., 2021. Microfluidic Organs-on-a-Chip for Modeling Human Infectious Diseases. Accounts of Chemical Research, 54(18), pp.3550-3562. https://pubmed.ncbi.nlm.nih.gov/34459199/
Wouters, O., McKee, M. and Luyten, J., 2020. Estimated Research and Development Investment Needed to Bring a New Medicine to Market, 2009-2018. JAMA, 323(9), p.844. https://jamanetwork.com/journals/jama/fullarticle/2762311
Wu, Q., Liu, J., Wang, X., Feng, L., Wu, J., Zhu, X., Wen, W. and Gong, X., 2020. Organ-on-a-chip: recent breakthroughs and future prospects. BioMedical Engineering OnLine, 19(1). https://biomedical-engineering-online.biomedcentral.com/articles/10.1186/s12938-020-0752-0
Disclaimer: The views expressed here are those of the author expressed in their private capacity and do not necessarily represent the views of AZoM.com Limited T/A AZoNetwork the owner and operator of this website. This disclaimer forms part of the Terms and conditions of use of this website.