Discover the basics of utilizing FTIR spectrometry in gas detection, analysis, monitoring, and testing.
Could you briefly explain FTIR technology and how it is applied in gas detection?
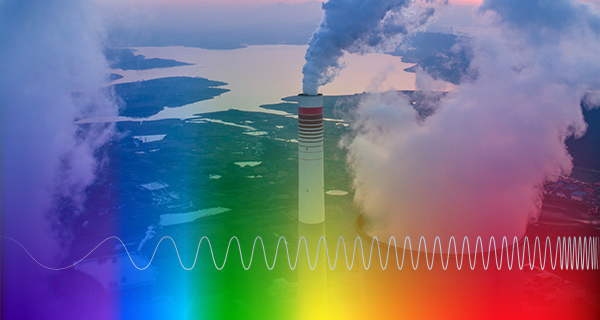
Image credit: Thermo Fisher Scientific
For more details on FTIR (Fourier Transform InFrared Spectrometry), please check out my webinar. I will talk about the basics of FTIR as well as our enhanced FTIR, Thermo Scientific StarBoost™.
FTIR is a technique for detecting and analyzing gases based on how they absorb infrared light. Most gases absorbs infrared light at precise wavelengths, and these absorption patterns act like fingerprints, allowing us to identify and quantify these gases.
In FTIR, the infrared light is analyzed using a device known as a Michelson interferometer. The interferometer creates an optical interference pattern called the interferogram. The interferogram is then transformed into a "single beam" spectrum using Fourier analysis. Ratioing the single beam spectrum from a blank sample (usually nitrogen gas which does not absorb infrared light) to the single beam spectrum (which contains the gases of interest) forms the transmission spectrum of the gases. This spectrum shows which gases are present and in what amounts.
FTIR is commonly used because it can detect many gases simultaneously and with great precision. However, as with any analytical measurement, measurement noise can limit its sensitivity, which is where advanced systems such as the Thermo Scientific™ MAX-iR™ FTIR Gas Analyzer utilizing optically enhanced Thermo Scientific StarBoost™ technology comes into play.
What is StarBoost technology? How does it compare to conventional FTIR technologies?
The StarBoost system builds upon the core principles of FTIR technology but enhances it in several significant ways. StarBoost outperforms typical FTIR systems in terms of sensitivity and detection limitations. Our typical Max-iR DTGS system operates within a wave number range of 500 to 5,000. However, with StarBoost, we apply a long-pass optical filter to cut down the spectrum, focusing on wave numbers between 1,800 and 3,400.
This shorter range, along with increased signal and signal-to-noise ratio (SNR), enables us to identify gases at concentrations as low as single-digit parts per billion or even parts per trillion. In one of our experiments, we were able to detect carbon dioxide at a concentration of 10 parts per trillion in bulk nitrogen. That degree of sensitivity was previously impossible with conventional FTIR instruments.
Could you please describe the essential components of the StarBoost technology and how they improve gas detection capabilities?
StarBoost comprises many important components that work together to greatly increase the sensitivity of our gas detection systems. The first critical component is the high D-star (or high-sensitivity) detector, which is thermoelectrically cooled. Unlike prior systems that used liquid nitrogen cooling, we switched to this more effective technology, which makes the system easier to handle and compact.
We also use an optical filter system, which contains a bandpass filter that limits the useable infrared radiation to a specified region to insure that the response of the detector signal is linearly proportional to the light striking it. We also modified the circuitry, using low-noise electronics with increased amplifier gain and a revolutionary auto-referencing software. These components improve the system’s capacity to detect gases at extremely low concentrations, down to the part-per-trillion level.
What role does the high-definition star detector play in the StarBoost system, and why is thermoelectric cooling relevant in this context?
The high D-star detector lies at the heart of StarBoost technology. It is a mercury cadmium telluride (MCT) detector that is extremely sensitive and responds to a wide range of infrared wavelengths.
The thermoelectric cooling method is important because it eliminates the requirement for liquid nitrogen, which was previously utilized to cool these detectors. Using thermoelectric cooling, we can retain high sensitivity while decreasing operational complexity.
The cooling reduces thermal noise, which is important when trying to detect gases at parts-per-billion or even parts-per-trillion levels. However, the detector’s active sites can become saturated at high light intensities. Therefore, we linearize the response to prevent signal saturation, as was mentioned previously.
How does linearization influence the detector’s absorption signal? Could you explain how this increases sensitivity?
The linearization process is important in boosting sensitivity, especially when working with quantum detectors such as the MCT detector. At high light intensities, the detector’s active sites can saturate, which means that no more signal is created beyond a certain point, regardless of how much more light is delivered. This is a non-linear response, which reduces the system’s capacity to detect small signal changes.
By lowering the light intensity and working within the detector’s linear range, we ensure that even modest changes in light intensity result in proportionate changes in the signal. In other words, a 10 % decrease in light intensity causes a 10 % change in the detector’s response. This increases the system’s sensitivity, allowing us to detect many tiny signals that would otherwise be overlooked.
How did you improve the signal using amplifier gain, and how did this affect your prototype development?
We originally exhibited the StarBoost concept in 2016 with a promising prototype. In that prototype, we employed a high D-star detector and optical filters to boost the signal. Initially, without altering the amplifier strength, we saw a three-and-a-half-fold increase in signal simply by introducing a filter into the optical path.
The big breakthrough occurred when we increased the amplifier gain. By doing so, we amplified the signal by a factor of 30, making a significant effect. The prototype allowed us to demonstrate how much more sensitive the system could become using this mix of strategies. Essentially, we went from being dominated by electrical noise to being detector noise limited, substantially decreasing our detection limits while increasing overall sensitivity.
Watch the webinar on-demand
How does the StarBoost technology increase the SNR ratio, and what specific adjustments did you implement?
The improved SNR was the direct outcome of numerous combined breakthroughs in StarBoost technology. First, by using an optical filter to linearize the detctor signal. Then, we raised the amplifier gain, which enhanced the signal. This enabled us to enhance even tiny signals without generating excessive noise.
We achieved a considerable gain in SNR by working in a detector noise-limited environment rather than one bound by electrical noise. For example, in our StarBoost testing, we witnessed a signal boost of 40 times that of our normal Max-iR system, resulting in a 40-fold gain in SNR.
What is auto-referencing, and how does it help yield more sensitive FTIR measurements?
Auto-referencing is a revolutionary data processing approach that was developed to improve the sensitivity of FTIR measurements. The premise is simple yet quite effective. We begin by obtaining a typical interferogram 1024 points with a resolution of two wave numbers. Then, we truncate the same interferogram to 256 points, increasing the resolution to eight wave numbers.
When we calculate the ratio of these two signals—the standard resolution signal and the lower resolution background—we effectively remove any wide spectral characteristics and baseline shifts. This gives us a absolutely flat baseline which allows us to detect significantly smaller absorbances. The absorbances in this technology are directly proportional to gas concentration, making it possible to detect even extremely low concentrations with excellent precision.
About the interviewee

Dr. Charles M. (“Mark”) Phillips is a Senior Applications Engineer at Thermo Fisher Scientific Gas Analysis Solutions. He provides application and technical support on Thermo Fisher’s MAX-iR™ FTIR product line.
Dr. Phillips has had extensive experience in the spectroscopic detection of gases, with technical positions at Scott Specialty Gases (now AirGas/Air Liquide Specialty Products) and MKS Instruments/OnLine Products. Dr. Phillips received his B.S. in Chemistry from Oakland University, and his Ph.D. degree in Physical/Analytical Chemistry from Michigan State University. He also accepted a postdoctoral research position at the Massachusetts Institute of Technology and was the associate director of the regional laser and biotechnology laboratories at the University of Pennsylvania.
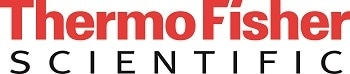
This information has been sourced, reviewed, and adapted from materials provided by Thermo Fisher Scientific – Environmental and Process Monitoring Instruments.
For more information on this source, please visit Thermo Fisher Scientific – Environmental and Process Monitoring Instruments.
Disclaimer: The views expressed here are those of the interviewee and do not necessarily represent the views of AZoM.com Limited (T/A) AZoNetwork, the owner and operator of this website. This disclaimer forms part of the Terms and Conditions of use of this website.