A unique facility is devoted to the research and development of novel and pioneering radiation detector technologies at Kansas State University.
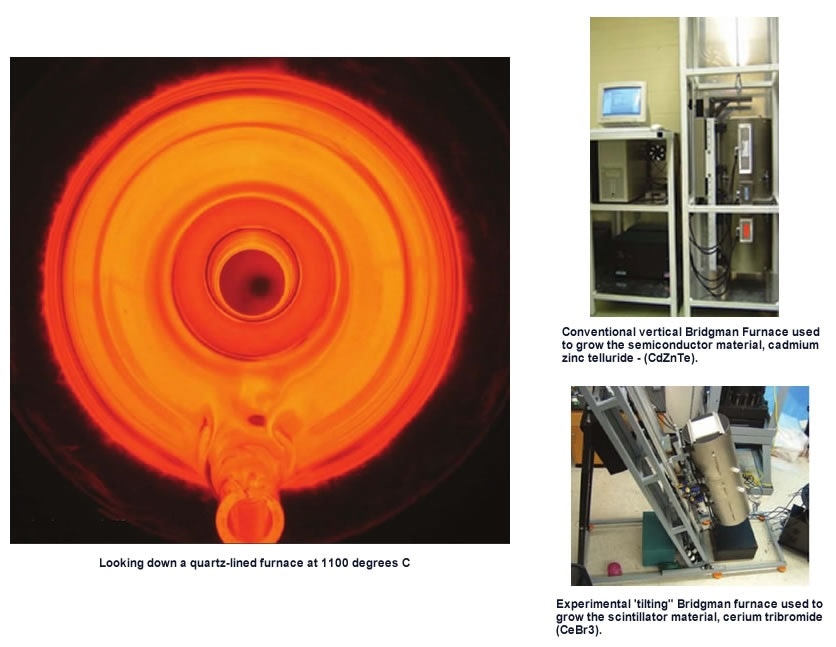
The Semiconductor Materials and Radiological Technologies (SMART) Laboratory is the biggest radiation detection laboratory in the United States that is university-based. It centers on the detection of gamma rays and neutrons, mostly those from special nuclear material (SNM) for homeland security applications.
The SMART Lab analyzes and fabricates various detectors, which comprise miniature gas-filled detectors, pixelated devices for neutron or gamma-ray imaging, high-resolution, room-temperature-operated semiconductor gamma-ray spectrometers, and compact low-power neutron detectors.
The laboratory manufactures detectors from start to finish in readily deployable packages for utilization in the detection of nuclear materials such as uranium and plutonium.
The gamma-ray detection aspect of the laboratory's research centered on the development and discovery of dense, high-Z semiconductor material, for example, cadmium zinc telluride (CdZnTe or CZT) and mercuric iodide (HgI2), and multiple scintillator materials such as lanthanum (LaBr3) and cerium tribromide (CeBr3).
Research performed on large crystal growth with scintillator materials and high-Z semiconductors has created large crystal ingot yields.
Gamma rays are electromagnetic radiation of high frequency, with a very short wavelength, that is generated by subatomic particle interactions, for example, fission, fusion, radioactive decay, and electron-positron annihilation.
Gamma radiation is highly penetrating photons and is very energetic. To specifically detect them is highly complex, but a material with a high Z number is required, signifying a great number of protons and neutrons in the nucleus.
Those nuclei tend to stop gamma rays more efficiently than different elements such as helium or hydrogen. A crystal with a high-Z number changes the gamma rays from electromagnetic waves into excited electrons.
The electrons create light or move through the crystal and make something that can be detected. If a crystal is highly homogeneous and uniform, it can be known that a gamma-ray interacted with the crystal by the influence that is observed in it.
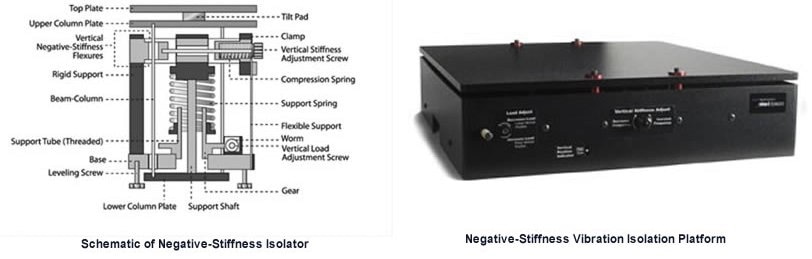
Growing Giant Crystals
Crystals of CdZnTe and the scintillator materials are grown using a vertical Bridgman furnace at the SMART Laboratory. In this method, molten material is directionally solidified from one end to the other to create a large-volume ingot in the form of a single crystal.
Techniques to grow CdZnTe for gamma-ray spectrometers have been discovered since the early 1990s, but an effective system to create large crystals cost-effectively has not been attained until relatively recently.
Higher ingot yields produce more accurate, smaller, and faster sensors, and enable gamma-ray detectors to be more economical and portable in the field. These benefits can have an extensive impact on the objectives of national security. Radiation detectors employing CZT can function in direct-conversion (or photoconductive) mode at room temperature.
The SMART Lab researchers encapsulate the material to be grown within a quartz ampoule in vacuum conditions. The quartz tube is placed inside the furnace vertically, changing the material to a molten state of between 500 - 1,100. They then steadily freeze the material from bottom to top.
If the thermal gradients are adequately carried out, a large crystal will be produced. Once it has grown, the crystal is extracted from the tube and is trimmed to size with a diamond saw. It is then polished to create a detector.
Vibration Isolation
The maximizing stable crystal growth process through the elimination of external vibrations is essential to optimizing ingot yield.
The general understanding within the crystal growth community is that uncontrolled vibrations can cause the growth interface to destabilize.
Professor Mark Harrision at SMART lab explains, "As the material is freezing from bottom to top, there is an interface between liquid and solid, and it sets up a natural convection flow that is ideal for growing a big, single crystal.”
He continues, “If a vibration disturbs the liquid directly above the forming crystal solid, it can change the convection patterns and multiple crystals will form from the previous single crystal. Which is contrary to our purpose of growing large, single crystals.”
"We looked into various active and air-table vibration handling systems, and eventually selected negative-stiffness vibration isolation," he adds.
Negative-stiffness mechanism (NSM) isolators have the flexibility of the custom tailoring of resonant frequencies horizontally and vertically. They utilize an entirely mechanical concept in low-frequency vibration isolation.
Vertical-motion isolation is provided by a stiff spring that supports a weight load in combination with an NSM. The total vertical stiffness is very low without influencing the static load-supporting ability of the spring.
Horizontal motion isolation is provided by beam-columns connected in series with the vertical-motion isolator. The horizontal stiffness of the beam-columns is decreased by the ‘beam-column’ effect.
A beam-column performs as a spring in combination with an NSM. The outcome is a tightly packed, passive isolator capable of very low horizontal and vertical natural frequencies and very high internal structural frequencies.
Vibration transmissibility with negative-stiffness is significantly enhanced compared to air systems, which can make vibration isolation issues increase because they contain a resonant frequency that can reflect that of floor vibrations.
Transmissibility is a measure of the vibrations that transmit through the isolator in relation to the input vibrations. The Negative-Stiffness isolators, when modified to 0.5 Hz, attain a 93% isolation efficiency at 2 Hz; 99% at 5 Hz; and 99.7% at 10 Hz.
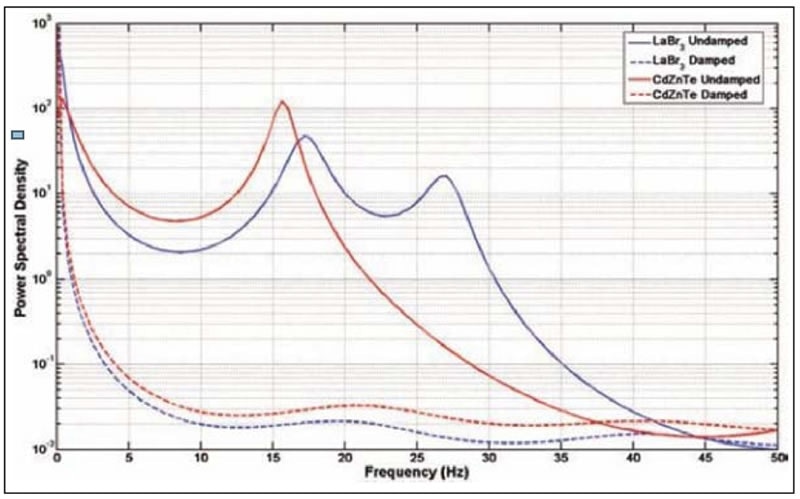
Plots of power spectral density measurements made with a seismometer at the growth ampoule position in the two furnances, with and without the negative-stiffness isolation platform.
NSM transmissibility is also enhanced compared to active systems. As they are powered by electricity, active systems can be negatively affected by issues of power modulations and electronic dysfunction, which can disturb the continuity of crystal growth.
Active systems also have a restricted dynamic range, which is easy to exceed and results in the isolator going into positive feedback and producing noise beneath the equipment. Active isolation systems have essentially no resonance, as their transmissibility does not roll off as quickly as negative-stiffness isolators.
"One of the concerns we had was surface waves coming across the ground, which induce vibration in the crystal growth system," outlines Professor Harrison.
"We are located in a basement," he adds, "Before we got the NSM system, I could actually see somebody walking down the stairs through the walls with a seismometer. With the negative-stiffness system in place, I can't even tell when they are shelling at the nearby Fort Riley military base."
Improving Detection
Gamma-ray detectors have been present for many years, but they either require liquid nitrogen cooling, such as those using germanium, or have a very low efficiency and performance. The complexity needed to take liquid nitrogen into a remote desert to discover special nuclear material, for example, is challenging.
"What we are trying to do at SMART Lab is make it more feasible, more economical for these detectors to be put in place at every critical check point, at every airport and shipping port," explains Professor Harrison. "This will increase the possibility of detecting and intercepting shipments of special nuclear material, should they occur."
Acknowledgments
Produced from materials originally authored by Jim McMahon from Minus K.
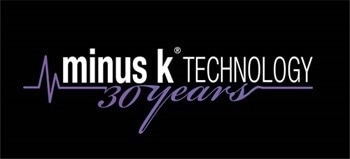
This information has been sourced, reviewed, and adapted from materials provided by Minus K Technology.
For more information on this source, please visit Minus K Technology.