Introduction
Since their discovery by Wöhler in 1830 the tungsten bronzes have been extensively studied due to their interesting chemical, electrical and optical properties [1-2]. The well known ReO3-type structure characterises many compounds within this extended family. The common structural feature of these materials is the WO6 unit which is repeated along three directions through shared corners. This structural arrangement provides empty tunnels, running parallel to the b-axis, where ions can be inserted to form a great variety of compounds called perovskite tungsten bronzes.
Different structural arrangements can be produced if some of the WO6 units are replaced by monophosphate (PO4) or diphosphate (P2O7) groups, and then a new compounds called phosphate tungsten bronzes are formed [3-6].
Recently in our group we have started a systematic study about the ability of these bronzes to insert small ions like lithium. Different features of these materials, i.e. a) its framework based in the perovskite type structure produces an open structure suitable for lithium insertion reactions, see Figure 1, and b) the high oxidation state of the transition metal that forms the framework, suggests a large amount of lithium may be inserted.
In the present work we have carried out an electrochemical lithium insertion study into some ternary oxides within the diphosphate tungsten bronzes with hexagonal tunnels (DPTBh). These bronzes are formed when the size of the guest cation, A, is larger or equal to that of K+ [7-8]. Stability phase domains of diphosphate tungsten bronzes AxP4W8O32 (A = K, Na) have been studied previosuly by X-ray diffraction [9]. In this sense, we have selected four diphospahte tungsten bronzes belonging to the phase diagram AxP4W8O32 (A = K, Na; x = 0.8, 1.5) in order to check their potential as cathodes in lithium batteries.
Experimental
Several samples of the diphosphate tungsten bronzes, AxP4W8O32 (A = K, Na; x = 0.8, 1.5), were obtained in two steps. In the first instance, a mixture of (NH4)2HPO4, WO3 and Na2CO3 (or K2CO3) in the appropriate molar relation was heated in air at 650 oC for 24 h in order to decompose the ammonium phosphate [10]. Then, a stoichiometric amount of W metal was added to the starting mixture to reach the composition AxP4W8O32 (A = K, Na; x = 0.8, 1.5). After grinding, the mixture was placed in a quartz tube that was evacuated, sealed and heated at 1000oC for a week and then slowly cooled to room temperature [11] Structural characterization was carried out by X-ray diffraction using a Siemens D-5000 diffractometer with Cu Kα (λ= 1.5418 Å) radiation. X-ray diffraction data of the pristine oxide were collected between 2θ range of 5 to 90º with a scan rate of 0.05º/2s.
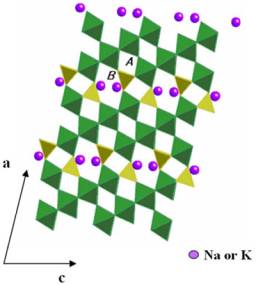
|
Figure 1. Structural crystalline of the bronze A1.5P4W8O32 along the b-axis. Two type of tunnels are present: A) quadrangular and B) hexagonal.
|
The electrochemical insertion of lithium was studied by means of an electrochemical cell of the Swagelok type bearing metallic lithium as the negative electrode. The positive electrode was a 7 mm diameter pellet obtained by pressing 15-25 mg of a mixture containing ternary oxide, carbon black and ethylene-propylene-diene-terpolymer (EPDT) in a 89:10:1 ratio. For the electrolyte, a 1 mol dm-3 solution of LiPF6 in a mixture of ethylene carbonate (EC) and dimethyl carbonate (DMC) 50:50 was used. Due to the high reactivity of metallic lithium, the assemblage of the cells were carried out in an argon filled glove box (MBraun) with a content of oxygen and water less than 1 ppm. The assembled cell was then removed and connected to a multichannel galvanostatic-potentiostatic system (MacPile II). In typical potentiostatic experiments a voltage scanning rate of +10 mV/h and +10 mV/0.5 h were applied to cycle the cells between different voltages vs Li+/Lio. When cells were discharged galvanostatically current of densities of 80 and 160 μA/cm2 were applied.
Results and Discussion
The solid state reaction between the reactant oxides produced a blue powder for all compositions prepared with formula AxP4W8O32 (A = K, Na; x = 0.8, 1.5). In each case the corresponding X-ray diffraction pattern showed good agreement with that reported previously. On the basis of these results we tested the four compositions prepared for their electrochemical lithium insertion reactions.
When several cells with configuration Li/electrolyte/AxP4W8O32 were charged-discharged between 3.0-0.5 V vs Li+/Li0 under galvanostatic conditions, the variation of the voltage with respect to the amount of lithium inserted showed a behaviour as is observed in the Figure 2.
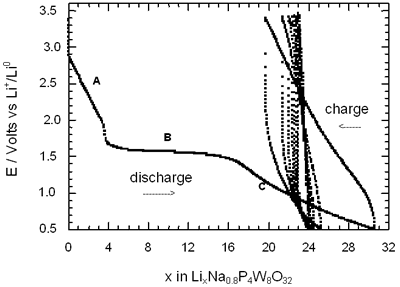
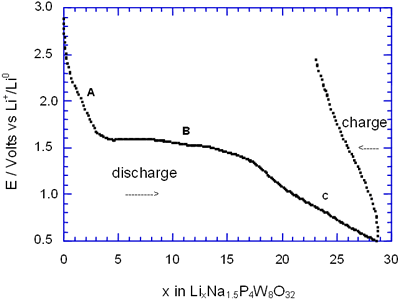
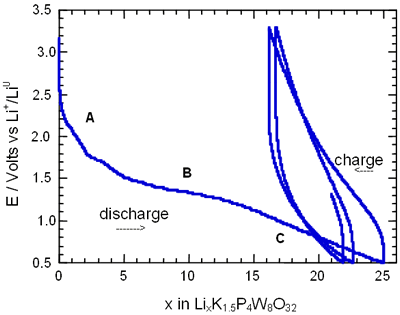
Figure 2. Voltage-Composition plot for several charge-discharge cycles of cells with configuration Li/electrolyte/AxP4W8O32 under galvanostatic conditions.
In general, all compositions studied exhibited a similar behaviour featured mainly by a large semiconstat potential region located around 1.5 V vs Li+/Lio. In the same way, during discharge of the cells two regions were easily detectable where the voltage drops abruptly in relation to the amount of lithium inserted. These regions labelled as A and C were associated with a solid solution in each case. The maximum amount of lithium inserted under these conditions, approximately 30 lithium atoms per formula unit, leads to a high specific capacity of 380 Ah/Kg. Nevertheless, after the first cycle the electrochemical system was unable to maintain the high specific capacity of the cell due to the irreversible process labelled as B. In order to check this situation we have carried out charge-discharge cycles of the cells Li/electrolyte/AxP4W8O32 just in the region associated with the process labelled as A. Figure 3 shows E-composition plot for the sodium bronzes NaxP4W8O32 (x = 0.8 and 1.5) where it can be observed that the origin of irreversibility is associated with process B (A → C). A similar situation was observed for the potassium bronzes KxP4W8O32 (x = 0.8 and 1.5).
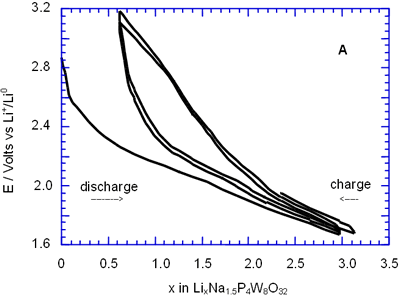
Figure 3. Voltage-Composition plot for a charge-discharge cycle of two cells with configuration Li/electrolyte/NaxP4W8O32 when it were cycled until 1.8 V vs Li+/Lio.
In order to know more about the nature of the different process observed along the charge-discharge of the cells Li/electrolyte/AxP4W8O32, we have followed the kinetics of the lithium insertion through the relaxation curves I(t). In all cases, for potential values around the peak labelled as B, the behaviour of current with time is far from a t-1/2 relationship [12], see Figure 4.
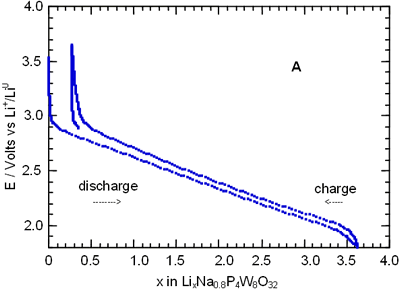
Figure 4. Chronoamperogram obtained by discharging a cell Li//Na1.5P4W8O32 at a scan rate of - 10 mV/ h.
Hence we have deduced that the diffusion of the lithium ions is not the process that limits the reaction. This behaviour indicates that the system is crossing a two-phase domain. In this case, the shape of the peak “B” in I-E diagram is asymmetrical with a linear slope whose intercept with the zero current is at the open-circuit two-phase equilibrium value. Similar behaviour has been observed for all bronzes studied showing that the lithium insertion mechanism is similar for each phase.
Figure 5 shows the complete charge-discharge of the cell Na1.5P4W8O32 through its I-E curve. In general, the presence of the peak labelled as B was associated with the large semiconstant potential region detected in the correspondence E-composition plot. Note that during the charge process, i.e. oxidation, peak B is missing after the first cycle in Na1.5P4W8O32 due to its irreversible nature.
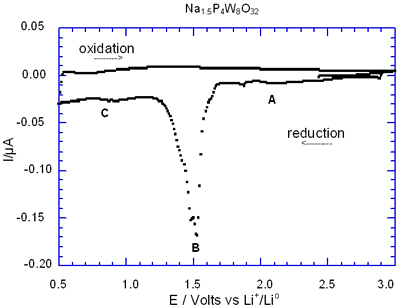
Figure 5. Voltamperogram obtained by discharging the cell Li/electrolyte/Na1.5P4W8O32 at a scan rate of -10 mV/ 0.5h.
The average lithium inserted in the four diphosphate tungsten bronzes AxP4W8O32 was around 30 lithium per formula, leading to a specific capacity of the cell of 380 Ah/kg. Although this value is high, after the first cycle approximately 25-40 % lithium inserted can not be removed.
The crystal structure of AxP4W8O32 phases (A= Na and K) is similar to that of P4W8O32. In bronzes with higher ion concentration, see Figure 1 for A1.5P4W8O32, pseudohexagonal tunnels are formed in which A+ ions are located. On the other hand, empty quadrangular tunnels also exists. In AxP4W8O32, (A = Na and K) four quadrangular and one pseudohexagonal tunnels are present. Taking into account that AxP4W8O32 can insert up to approximately 25-30 lithium per formula, a multioccupancy of the tunnels is necesary to justifity such an inserted amount.
Conclusions
An electrochemical study of the lithium insertion in Ax(PO2)4(WO3)8 (A= Na, K; x= 0.8 and 1.5) through potentiostatic and galvanostatic experiments was carried out. Due to the high oxidation state of the W in the oxides the amount of lithium inserted in alkali phosphate tungsten bronzes was large, but due to irreversible processes, specific capacity of the cells were dramatically lost after the first cycle. Taking this behaviour into account we suggest that this bronzes only can be considered as a cathode in primary lithium batteries.
Acknowledgements
We wish to thank to CONACYT for supporting the project 43800 and the Universidad Autónoma de Nuevo León (UANL) for its invaluable support through the project PAICYT CA839-04.
References
1. P. Hagenmuller, “Tungsten bronzes, Vanadium bronzes, and related compounds”, in Comprehensive inorganic chemistry, ed. Pergamon, Oxford, 1973, p. 541.
2. M. S. Whittingham, “Intercalation chemistry: an introduction” in Intercalation Chemistry, ed. Academic Press, New York, 1982, p. 8.
3. E. Canadell and M. H. Whangbo, “On the possible electronic instability of the monophosphate tungsten bronze (WO3)4(PO2)4”, J. Solid State Chemistry, 86 (1990) 131-134.
4. E. Canadell , M. H. Whangbo and I. E. Rachidi, “Similarity of the electronic properties of the monophosphate tungsten bronzes”, Inorg. Chem., 29 (1990) 3871-3875.
5. M. Greenblatt, “Phosphate tungsten bronzes: a new family of quasi-low-dimensional metallic oxides”, International Journal of Modern Physics, B7 (1993) 3937-3971.
6. P. Roussel, P. Labbé and D. Groult, “Symmetry and twins in the monophosphate tungsten bronze series (PO2)4(WO3)2m (2 < m < 14)”, Acta Crystallographica, B56 (2000) 377-391.
7. J. P. Giroult, M. Goreaud, Ph. Labbé and B. Raveau, “Bronzes with a tunnel structure RbxP8W8nO24n + 16. III. RbxP8W28O100 : a member corresponding to a non-integral n value n = 3.5”, Acta Crystallographica, B38, (1982) 2342-2347.
8. M. Lamire, Ph. Labbé, M. Goreaud and B. Raveau, “Ba2P8W32O112: structural study in comparision with the K and Rb diphosphate tungsten bronzes with hexagonal tunnels”, J. Solid State Chemistry, 71 (1987) 342-348.
9. P. Roussel, D. Groult, A. Maignan and P. Labbé, “Phase relations, crystal structure, and electron transport properties of phosphate tungsten bronzes (KxNay)(PO2)4(WO3)2m (m = 4,6)”, Chem. Mater., 11 (1999) 2049-2056.
10. B. Domengés, M. Hervieu and B. Raveau, “Monophosphate tungsten bronzes with hexagonal tunnels, Nax(PO2)4(WO3)2m : X-ray diffraction and HREM study”, Acta Crystallographica, B46 (1990) 610-619.
11. B. Domengés, M. Hervieu and B.Raveau, “Potassium monophosphate tungsten bronzes with hexagonal tunnles, Kx(PO2)4(WO3)2m: X-ray diffraction and HREM study”, J. Solid State Chemistry, 72 (1988) 155-172.
12. Y. Chabre, “Step potential electrochemical spectroscopy of proton intercalation in γ/ε-MnO2”, J. Electrochem. Soc., 138, (1991) 329-330.
Contact Details
|