Surface energy is important in a number of industrial applications and processes. It shows a very strong dependency on several macroscopic properties and is related to a number of critical interfacial phenomena such as wetting behaviours and adhesion.
Since carbon nanotubes (CNTs) have generated a vast amount of interest in recent years, due to their electrical, structural and mechanical properties, the study of nanomaterials has largely increased. CNTs are sheets of graphene rolled into tubes and vary in terms of the number of carbon layers in the sidewall.
They can be single-walled, double-walled and multi-walled. CNTs are energetically inhomogeneous showing several surface sites, such as specific functional groups or structural defects. Hence, a surface energetic heterogeneity profile is capable of providing in-depth insights into the population and nature of these surface sites. This kind of a heterogeneity profile helps predict the characteristics of products, especially while formulating composites, blends or coatings.
Until now, the emphasis on characterization of the surface energy distribution of nanomaterials has been minimal. Latest developments in iGC surface energy methodology help determine surface energy distributions from the adsorption isotherms of a series of n-alkanes at finite concentrations.
This novel approach is adapted by iGC Surface Energy Analyzer (SMS, Alperton, UK) and is equipped with advanced injection technology, which helps accurately control the injection size. This article details the measurement of the surface energetic heterogeneity of commercial multi-walled CNTs, with respect to the effects of a range of modification treatments, such as oxidation and annealing.
Method
Material – Multi-walled Carbon Nanotubes
Multi-walled commercial CNTs (MWCNTs) were used during the study (Arkema SA, Lacq-Mourenx, France). The synthesis of these MWCNTs was done using chemical vapor deposition, with outer diameters of approximately 10-20nm and lengths of at least a few microns.
The received MWCNTs were modified using:
- High temperature annealing (treatment at 2100°C for 2h under argon flow; CNTs were cooled to room temperature under argon and then exposed to air)
- Thermal oxidation (short treatments at 640°C under air flow)
Traditional techniques such as electron microscopy, spectroscopy and thermal analysis were used to characterize both the surface modified CNTs and the as-received CNTs.
Surface Energy Heterogeneity
iGC SEA (SMS, Alperton, UK) was used for the analysis and the data were analysed using the standard SEA Analysis Software. 10mg of MWCNT samples were packed into individual iGC glass columns (300mm long by 3mm inner diameter).
In order to determine the dispersive surface energy distribution and the specific (acid-base) surface energy distribution, samples were run at a series of surface coverages with alkanes and polar probe molecules.
The Dorris and Gray method was used for the analysis for dispersive surface energy component. The specific contribution was obtained by first measuring the free energy of desorption of a pair of mono-functional acidic and basic probe molecules, based on the polarisation approach and Della Volpe scale.
In this study, four n-alkanes, nonane, octane, heptane and hexane and six polar probes-toluene, dichloromethane, ethyl acetate, chloroform, acetone and ethanol were used.
Each sample column was conditioned in-situ at 150°C and 0% RH with a helium carrier gas flow of 10sccm before every injection. All solvent injections were conducted at 100°C and 0% RH, using the same carrier gas flow rate. For dead volume corrections, inert methane gas was used.
Results
Effect of Different Modifications on MWCNTs
The TEM and SEM images of as-received, annealed and oxidised MWCNTs are shown in Figure 1. It can be observed that all three samples show similar diameters (10-20nm) and texture (unaligned, wavy, morphology), typical for commercially produced MWCNTs.
.jpg)
Figure 1. HRTEM and SEM images of MWCNTs; (a) as-received, (b) annealed and (c) oxidised
There is a difference in the local and chemical structural surface properties of modified and as-received MWCNTs as determined by traditional analytical methods as shown in Table 1.
Annealed MWCNTs show higher graphitic crystallinity, however, thermal oxidation increased the surface oxygen content, confirming that additional surface groups were introduced.
Table 1. General characterisation data for as received and modified MWCNTs
MWCNTs |
BET specific surface area [m2/g] |
Raman spectra – Crystallinity IG/ID ratio |
XPS – Surface oxygen content [atom %] |
As-received |
237 |
0.94 ±0.02 |
0.5 |
Annealed |
215 |
1.33 ±0.03 |
0.6 |
Oxidised |
256 |
0.89 ±0.03 |
1.4 |
Dispersive Surface Energy Heterogeneity
Figure 2 shows dispersive surface energy (γsD) profiles from which it can be seen that all MWCNT samples were energetically heterogeneous. The degree of energetic heterogeneity however, depended on the modification treatment with oxidised MWCNTs found to be energetically most active and most heterogeneous. Considerably high dispersive energies were observed in all MWCNT samples corresponding to a typical value for graphitic carbon materials.
It can be inferred from the results that the iGC technique can be used for determining very minute differences between the MWCNTs. It is also understood that energetic surface heterogeneity can have a significant impact on the thermodynamic characterisation of carbon surfaces.
.jpg)
Figure 2. Dispersive surface energy profiles.
To represent the heterogeneity of samples better, the surface energy distributions were obtained by a point-by-point integration of the surface energy profiles, resulting in plot of (γsD) versus percentage of surface (area increment), as shown in Figure 3.
.jpg)
Figure 3. Dispersive surface energy distributions
MWCNTs had (γsD) varying values from ~ 87 to ~107mJ/m2.
As the crystallinity of MWCNTs increased with thermal annealing, the annealed surface possessed only slight variations in (γsD) (~87 to ~95 mJ/m2). This implies a relatively homogeneous surface property.
At lower surface coverages (< 6%), the (γsD) values of as-received and annealed samples differ, but converge at higher surface coverages. This shows the importance of measuring surface energetic heterogeneity profile for real solids.
In contrast to the other two samples, oxidised MWCNTs had a much wider range of (γsD) values, varying from ~102 to ~155mJ/m2. This may be because of the introduction of additional surface functional groups with high (γsD), the creation of structural defects (such as micropores and graphene edges), and the opening of initially closed MWCNTs during the thermal oxidation process.
Please click here if you would like more information on the instrument used in this article or a quote
Surface Polarity and Acid-Base Surface Chemistry of MNCNTs
The acid-base (specific) surface energy (γsAB) profiles of all three MWCNT samples, determined from chloroform (monopolar acid) and toluene (monopolar basic) can be seen in Figure 4.
Although γsAB values were measured with just two monopolar probes, they offer a comparative assessment of the MWCNTs’ potential to undergo specific interactions.
.jpg)
Figure 4. Specific (acid-base) surface energy profiles.
Oxidised MWCNTs had fairly higher concentrations of Lewis acid-base functional groups on their surfaces, with nearly an 80% increment in γsAB values comparing to the as-received MWCNTs.
When compared to annealed MWCNTs, the γsAB of as-received MWCNTs was marginally higher. Also, the presentation of the surface polarity of these MWCNTs, in terms of percentage of γsAB, (Figure 5) revealed that the surface polarity of oxidised MWCNTs is the highest, followed by the as-received and annealed samples respectively.
.jpg)
Figure 5. Surface polarity (%) profiles.
With surface coverages, specific Gibbs free energy of desorption also changes, showing the heterogeneous nature of these samples. Oxidised MWCNTs especially, show stronger interactions with polar probes as shown in Figure 6, in agreement with their higher surface polarity.
Higher ΔGSP values are attributed to a higher concentration of polar surface groups or different surface groups with higher specific surface energy. All samples show a certain degree of interaction with all polar probes, however they predominantly interact with ethanol and acetonitrile probes.
Ethanol is slightly acidic and acetonitrile is slightly basic, and they are both bi-functional probes. Since the MWCNTs interact strongly with these bi-functional polar probes, this suggests that they are amphoteric in nature.
.jpg)
Figure 6. Specific free energy of desorption of six different polar probes with Oxidised MWCNT samples.
Surface chemistry of all MWCNTs was evaluated using the Gutmann acid (Ka) and base (Kb) numbers, identified based on the Gutmann concept. Values for each sample were calculated using the ΔGSP values of polar probes at that particular surface coverage.
Figure 7 shows the Kb (Lewis basicity) and Ka (Lewis acidity) profiles for all samples. It can be clearly observed from the results that Kb for all samples is consistently higher than Ka, indicating that the surfaces of these MWCNT samples are more basic in nature. This implies that sample surfaces have higher concentrations of electron-donating surface functional groups.
.jpg)
Figure 7. Gutmann acid and base numbers profiles.
It is apparent from the results that energetic heterogeneity and homogeneity of the as-received and surface modified MWCNT samples can be easily differentiated using IGC. This highlights the importance of determining any small differences in surface physical and/or chemical conditions of a wide range of solid materials.
Conclusion
IGC has been proven to be a sensitive and powerful technique for examining the surface chemistry and surface energy of carbon-based nanomaterials. This work shows the importance of ascertaining surface energies in dependence of coverage, such as through the measurement of surface energy profiles, for completely characterizing the difference in energetic heterogeneity of real solid surfaces.
With the help of such energy mapping techniques, IGC can show specific changes in surface properties, such as surface polarity or acid-base surface chemistry, which are not accessed readily by other traditional techniques, but are highly relevant to both the application and processing of CNTs. This work also shows the applicability of IGC technique to characterise a wide range of CNTs and nanomaterials having large accessible surface areas.
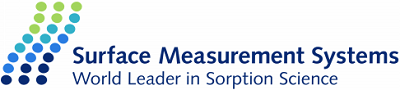
This information has been sourced, reviewed and adapted from materials provided by Surface Measurement Systems Ltd.
For more information on this source, please visit Surface Measurement Systems Ltd.