Polytetrafluoroethylene (PTFE) is an extremely popular and abundantly useful fluoropolymer. PTFE has been with ZEUS since the 1930s when it was accidently developed by Technicians working for DuPont. PTFE is considered to be unique as a fluoropolymer in that it is completely fluorinated comprising of only fluorine and carbon atoms, as shown in Figure 1.
This structure provides PTFE many desirable and unique properties. In fact, according to latest estimates, the global PTFE market is anticipated to reach USD 6.44 billion by the year 2020 presenting a combined annual growth rate of 8.1% [1]. This translates into an estimated global consumption of PTFE of 524.1 kilotons within the next three years [1]! PTFE is primarily known as Teflon® and is also known for its familiarity as a non-stick coating for cookware, but as this market overview shows, PTFE has indeed become a mainstay in many areas of the modern world.
.jpg)
Figure 1. Classical depictions of PTFE monomers shown in Lewis structure (left), bond-line drawing (center), and stereo representations (right).
Structure of PTFE
PTFE is a homopolymer of tetrafluoroethylene (TFE) and is produced by integrating tetrafluoroethylene monomers through a radical reaction (Figure 2). TFE polymerization is catalyzed by peroxide incorporated in the reaction, and the resulting reaction is self-sustaining until quenched or reactants are exhausted. The result of this reaction is long-chain (high molecular weight) molecules with considerable strength. PTFE chain length is extensively controllable and thus can be manipulated to suit particular applications. However, unlike most saturated carbons compounds, PTFE polymer chains are normally not flexible; they produce rigid rod-like molecules with unique chemical character.
.jpg)
Figure 2. General reaction scheme for the synthesis of PTFE.
PTFE’s unique properties stem from its multiple C–F bonds. Fluorine is known to have the highest electronegativity – its attraction for electrons including those involved in bonding – of any element on the periodic table [2, 3]. Carbon contains moderate electronegativity compared to fluorine, thus the C–F bond is greatly polarized towards fluorine. In addition to covalent sharing of electrons between fluorine and carbon, there are considerable electrostatic attractive forces drawing the partially positive carbon (Cδ+) towards the partially negative fluorine (Fδ–).
Thus, the covalent nature of the C–F bond together with its partial electrostatic character result in a C–F bond that is extremely short, and this effect is compounded with multiple C–F bonds in PTFE via multiple dipole interactions. C–F bond length in PTFE is usually on the order of 1.32 Å and for a C–F bond length in general is 1.35 Å [4]. This length is shorter than other carbon-halogen bonds and shorter than C–O and C–N bonds. (Only the C–Si bond is shorter than a C–F bond). The result is a close packing of PTFE along the C–C backbone, but in an unanticipated manner.
PTFE is a close-packed long-chain polymer. However, PTFE does not adopt the typical zig-zag pattern observed for most saturated carbon chains but is instead helical (Figure 3A). Generally, bigger substituents on adjacent carbons along a carbon chain backbone would place themselves in an anti conformation (Figure 4A). However, the fluorine atoms on adjacent carbons of PTFE cannot form a true staggered and anti conformation because of the large size of fluorine atoms and their high degree of electron density stemming from their electronegativity. Instead, the fluorine atoms position themselves along the carbon backbone in a helical conformation with dihedral angles along C–C atoms of slightly less than the 60° of a staggered anti conformation (Figures 3B and 4).
.jpg)
Figure 3. (A) Typical zig-zag conformation of a saturated hydrocarbon chain. (B) PTFE helical geometry with fluorine atoms surrounding the carbon chain backbone.
This rare phenomenon of PTFE is due not to the gauche effect proposed in small fluorinated alkanes but because of hyperconjugation and interaction of the C–C bonding and C–F anti-bonding orbitals (σCC→σ*CF) of PTFE (Figure 5) [5]. The decrease in energy due to hyperconjugation more than outweighs the energy penalty resulting from the smaller dihedral angle along adjacent C–C atoms. These energy benefits become more prominent with each additional –CF2–. The result is an even more closely packed helical PTFE with a superior degree of stability. This chosen helical conformation of PTFE over the zig-zag conformation is so stable that it has also been observed in perchlorinated alkanes [5]. (It should be noted that PTFE is present in at least two other phases or transitional conformations of changing degrees of helicity, based on pressure and temperature, of which Phase II dominates at approximate room temperature and below [6-8]. (These phases shall not be discussed here, however).
.jpg)
Figure 4. Newman projections: Geometry comparison of small fluorinated alkanes and PTFE. The staggered anti conformation of 1,2-difluoroethane, C2H4F2 (left), the gauche conformation of 1,2-difluoroethane (center), and the zig-zag staggered conformation of PTFE (right).
.jpg)
Figure 5. PTFE helical geometry illustrations: The exaggerated (quasi-) gauche conformation of PTFE (left), and same showing σCC→σ*CF orbital interaction and hyperconjugation (right).
Features and Properties of PTFE
PTFE’s close-packed nature and its completely bonded carbon chain give this polymer a multitude of desirable properties that have placed it in such demand today. Fluorine’s three lone pairs of electrons balance the overall dipole moment regardless of its C–F bond along with its high electron density. This refers to the fact that the fluorine atoms of PTFE are non-polarizable. The fluorine atoms of PTFE also have a complete valence shell octet of electrons. These two aspects render fluorines of PTFE poor electron donors and poor hydrogen bond acceptors. The result is an unreactive sheath of fluorine surrounding the C–C backbone of PTFE giving PTFE exceptionally good chemical resistance. (However, it is still possible for PTFE to be affected by specifically prepared conditions involving halogenated compounds or alkali metals).
The unreactive fluorine sheath of PTFE also has other important implications. PTFE’s outer compact fluorine “capsule,” with its high electron density, equally repulses other PTFE chains. As a result, PTFE surfaces have a very low coefficient of friction, usually reported in the range of 0.02 – 0.08. The unreactive nature of PTFE towards even small molecules such as water or other PTFE chains means that PTFE adheres to just about nothing. These features translate into extremely good lubricity for specified applications.
PTFE is also considered to be a rigid molecule. PTFE does not allow free rotation around the C–C backbone single bonds, unlike less halogen-saturated carbon chain polymers or simple hydrocarbon chains such as polyethylene. The electron density and the space needed for the electrons of the fluorine atoms results in steric repulsion via vicinal positions but also through the 1,3-positions. Such repulsions make the rotational energy barrier about the C–C single bonds greatly unfavorable, hyperconjugation notwithstanding. The result is a conformationally inflexible PTFE polymer chain.
PTFE Products and Catheter Componentry
It is possible to fabricate PTFE in a wide range of products. PTFE’s chemical and mechanical features allow these products to be used in a variety of applications. PTFE can be developed into molded parts; used as flexible pipe joints, electrical insulators, bearings, valve bodies, gears; and extruded as tubing (Figure 6). PTFE can be machined into precision parts with extremely fine tolerances and can be made into thin sheets or film and also heat shrink. PTFE’s tight-bonded nature displays itself in exceptional wear and abrasion resistance with ultimate lubricity.
.jpg)
Figure 6. Example PTFE extruded products: (A) unpigmented and (B) pigment tubing, (C) PTFE heat shrink with spiral pigmenting, (D) PTFE monofilament (close-up view), and (E) Zeus PTFE Sub-Lite-Wall® tubing. (Images not to scale).
With such properties as chemical resistance and lubricity, it is no wonder that PTFE found its way into medical applications where these features are particularly desirable. PTFE has a long history of successful and safe use in the medical industry dating back before the 1970s [9]. PTFE can be extruded as tubing with very thin walls making it perfect for vascular catheter componentry where uniform and small size diameters are paramount. PTFE can be used, for instance, for the inner wall (base liner) of guiding catheters in order to provide a remarkably smooth inner surface. The smooth PTFE inside diameter (ID) of these catheters lowers friction against different catheter technologies such as balloons, stents or atherectomy devices as they are pushed via the tight confines of the catheter lumen. If the catheter ID is not of sufficient lubricity, it is possible for devices such as stents to collapse in an accordion-like manner as they are being pushed via the catheter lumen. The effect of increased lubricity of the catheter ID is a reduced deployment force of catheter devices as they are passed through the lumen increasing the probability of a successful procedure.
Dip-Coating vs. Free extrusion for Catheter Base Liners
Dip coating is a competing technology for attaining thin polymer walls over a component. This process is performed in order to impart some surface attribute or quality to the coated part such as increased rigidness or lubricity. In the instance described here, dip coating can be performed onto a mandrel as part of the catheter construction process. Then, once the coating has cured, the additional components of the catheter, including nylon jacketing, braiding and a jacket re-flow process step are built upon the cured dip-coated mandrel (Figure 7). After completing the catheter construction, the mandrel is then removed from the newly built catheter leaving behind the dip-coated layer which becomes the innermost lumen wall.
.jpg)
Figure 7. Catheter construction: basic catheter components: mandrel, base linear, braiding reinforcement, jacket (nylon or Pebax® typical), and peelable (FEP) heat shrink.
Dip-coating, while appearing to be a simple process at the outset, has some limitations that prevent it from being commonly used for catheter construction. Dip coating can experience unevenness resembling the surface of orange peel. Sometimes, dip-coated surfaces can have the appearance of many cross-sectional lines called chatter resulting from vibrations during the coating process. Dip-coated surfaces can also have craters, depressions, or even holes in the cured layers caused by contaminants – including moisture – during the coating process. Surface defects of the catheter ID represent a grave impediment to catheter use. While these defects can be addressed in different ways, superior precision of the dip-coating process will typically entail greater cost and time.
Finally, also partly due to the defects described above, dip-coated mandrels for catheter ID lumen wall can experience poor adhesion. These deficiencies can be in the form of poor adhesion of the liner to the jacket laid down over the braiding or poor adhesion of the dip-coated layer (liner) to the mandrel itself. Imperfect adhesion can lead to delamination defects, the most serious defect for catheter liners. Such failure can affect the basic aspects of the catheter such as torsional transmittance, deflectability or pushability. The end result of such a defect is possibly a failure of the catheter itself. While rare in occurrence, delamination defects present a serious added risk regarding the dip-coating process.
Ultra-Small (Micro) Catheters and Their Applications
The demand for ever-smaller sized devices drives the pursuit of thin-walled extrusions and the dip-coating process for catheter construction. These devices, falling under the general description as micro-catheters, are required for navigating extremely small vasculatures for minimally invasive surgical procedures (MISPs). These procedures may be diagnostic, investigation, mapping or interventional in nature. Catheter design is balanced between functionality and construction. Fundamental targets focus on catheter trackability, flexibility, pushability and torqueability (transmittance or torsional force). Based on the tortuosity of vasculature being explored, catheter outside diameter (OD) and ID play a role in selecting a suitable design and device for the application. Thus, catheter ID wall thickness is a trade-off of the desired fundamental catheter functional attributes and application environment.
Zeus Sub-Lite-Wall® Streamliner™ Catheter Liner
Advancing micro-catheter designs to meet the requirements of cardio, neurovascular and peripheral markets is an overwhelming task. The demand for more functional and smaller devices is continuous. In light of these requirements, Zeus Industrial Products Inc. is manufacturing their new Sub-Lite-Wall® StreamLiner™ series of thin-walled catheter liners. Zeus Sub-Lite-Wall® tubing, already reaching wall thickness to 0.005" (0.127 mm) and below, has presently been made even smaller with StreamLiner™ series of thin-walled catheter liner extrusions. These specialty extrusions are produced from PTFE and build upon the long-standing success of Sub-Lite-Wall® products from Zeus. These thin-walled extrusions were primarily produced to give medical device manufacturers and engineers greater access to the body’s tortuous pathways. Now, with the state of this market driving access to smaller and smaller vasculatures, Sub-Lite-Wall® StreamLiner™ series of PTFE extrusions is all set to become the market standard.
The first offering from Zeus in the StreamLiner™ series, StreamLiner™ XT, improves upon its already existing best-in-class thin-walled catheter liner. StreamLiner™ XT, featuring Zeus’ LoPro™ proprietary technology, possesses a maximum wall thickness of 0.00075" (0.01905 mm) and can be developed with IDs as low as 0.013" (0.330 mm) (Figure 8). These super-small walled catheter liners allow for smaller finished device outside diameters (ODs) while also producing increased catheter lumen potential. PTFE’s high lubricity is of specific advantage, too, since it results in improved (decreased) deployment force within the catheter lumen for the technology (fiber optic, camera, etc.) that is being pushed through it.
.jpg)
Figure 8. Sub-Lite-Wall® StreamLiner™ XT: (A) StreamLiner™ XT extruded tubing, and (B) schematic, graphic illustration, and feature description of StreamLiner™ XT.
As a free extrusion, StreamLiner™ XT has a number of advantages over dip-coating. These PTFE liners are tougher and stronger when compared to conventional liners. StreamLiner™ XT does not experience the craters, depressions or holes to which dip-coated liners are susceptible. Thus, the uniformity of StreamLiner™ XT contributes to its super-smooth lumen ID surface finish. StreamLiner™ series of liners are also not prone to delamination which is associated with dip coated liners. Together, these improvements allow the Sub-Lite-Wall® StreamLiner™ series to reduce risk to patients while providing a great performance and effective alternative to dip-coating for successful patient outcomes.
Conclusion
PTFE is a synthetic fluoropolymer initially discovered in the 1930s which has grown into a global industry. PTFE’s fully fluorinated nature contributes to this polymer’s unique helical structure producing a wide range of beneficial properties. With such favorable properties such as low coefficient of friction and superior chemical resistance, PTFE made its way into the medical sector and has a long-established history of successful use there. Zeus Industrial Products Inc., in order to improve upon PTFE extrusion technology, has developed an ultra-thin-walled PTFE catheter liner introduced as its Sub-Lite-Wall® StreamLiner™ series.
The first offering from this series and featuring Zeus’ proprietary LoPro™ technology, StreamLiner™ XT, enhances upon Zeus’ already best-in-class wall thickness to create a liner with < 0.00075" (0.01905 mm) wall thickness. As a free extrusion, the StreamLiner™ series eradicates many of the risks of a competing technology, dip-coating, such as uneven lumen wall surface and delamination. These liners make for a more robust or sturdier finished device while retaining sufficient functional properties such as torqueablity, flexibility and pushability. The super lubricious catheter lumen ID significantly improves deployment force via the catheter for successful MISPs. Sub-Lite-Wall® StreamLiner™ XT permits greater access to small vessels including peripheral and neurovasculatures and also represents a new tool for medical device clinicians and engineers to enhance patient outcomes.
References:
- Polytetrafluoroethylene (PTFE) Market Worth $6,440.0 Million By 2020. In. Online: Grand View Research; 2015.
- Pauling L: The chemical bond: a brief introduction to modern structural chemistry: Cornell University Press; 1967.
- Pauling L: The Nature of the Chemical Bond and the Structure of Molecules and Crystals: An Introduction to Modern Structural Chemistry: Cornell University Press; 1960.
- O'Hagan D: Understanding organofluorine chemistry. An introduction to the C-F bond. Chemical Society Reviews 2008, 37(2):308-319.
- Cormanich RA, O'Hagan D, Bühl M: Hyperconjugation is the Source of Helicity in Perfluorinated n-Alkanes. Angewandte Chemie International Edition 2017:n/a-n/a.
- Tanaka H, Takemura T: Studies on the High-Pressure Phases of Polyethylene and Poly(tetrafluoroethylene) by Raman Spectroscopy. Polym J 1980, 12(6):355-361.
- Clark ES: The molecular conformations of polytetrafluoroethylene: forms II and IV. Polymer 1999, 40(16):4659-4665.
- Wang C, Duscher G, Paddison SJ: Electron energy loss spectroscopy of polytetrafluoroethylene: experiment and first principles calculations. Microscopy 2014, 63(1):73-83.
- Laustriat S, Geiss S, Becmeur F, Bientz J, Marcellin L, Sauvage P: Medical history of Teflon. Eur Urol 1990, 17(4):301-303.
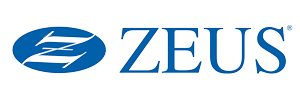
This information has been sourced, reviewed and adapted from materials provided by Zeus Industrial Products, Inc.
For more information on this source, please visit Zeus Industrial Products, Inc.