Remote plasma cleaning is a proven method used by several electron microscopists to get the most ideal images from their instruments by removing hydrocarbon (HC) contamination1. The oxygen radicals made in the plasma react with carbon compounds, generating H2O, CO, and CO2, which are removed from the instrument. According to quantum chemistry rules concerning energy loss, these oxygen atoms do not respond with diatomic molecules in two-body collisions, but they need a third body to dynamically remove excess energy. In SEM vacuum chambers, oxygen radicals can respond on chamber walls and other metal surfaces where they can be lost or recombine at pressure-dependent recombination rates.
XEI Scientific has carried out many studies2-4 employing experimentally-contaminated quartz crystal microbalances (QCM) in order to determine cleaning rates, demonstrating highly effective removal of hydrocarbons (Figure 1). Rapid decontamination rates were recorded in FIBs and SEMs supplied with turbo molecular pumps5, 6. At lower pressures, the longer means free path and reduced gas density result in higher cleaning effectiveness because of fewer radicals lost by collision (Figure 1). In addition, longer means free paths and lower pressures allow a flowing afterglow to form outside of the remote plasma radical source where metastable species decay, releasing ultraviolet and visible photons. Then, this afterglow light is observed to form in the full volume of the SEM and illuminates all surfaces. When air is used as the plasma source, a nitrogen afterglow with a distinct violet color is seen from the decay of N2+ metastables. A series of neutral nitrogen metastables generate UV lines at shorter wavelengths, and this adds energy to chamber surfaces to initiate hydrocarbon disassociation and desorption, causing oxidation by the reactive O radicals. This procedure accelerates the plasma cleaning activity and subsequent pump down. This procedure has been studied with an RGA (residual gas analyzer), quartz crystal monitors (QCM), and a spectrometer to demonstrate almost the entire removal of hydrocarbon residues from the chamber.
A recent study quantified the positioning of the plasma radial source and the effects of chamber geometry on cleaning efficiency. The study also demonstrated the advantage of plasma cleaning to develop hydrocarbon-free sample surfaces for the best possible data collection in EBSD/TKD experiments.
.jpg)
Figure 1. Hydrocarbon removal rate vs gas pressure at 20 Watts of RF power delivered to the plasma. Pressures above 100 mTorr were achieved using only a roughing pump, while a turbo molecular pump was used for pressures <100 mTorr to run in the Turbo Plasma cleaning mode.
Theory
In downstream or remote plasma cleaning, the oxygen radicals are developed and fill the vacuum chamber in the form of excited plasma. Air is the process gas in Evactron® Plasma Cleaning. A flowing UV afterglow is observed from the excited metastable nitrogen molecules with a typical pink/violet color at low pressures. The neutral radicals’ concentration in the plasma flowing UV afterglow is a function of the loss rate in the neutral afterglow and the production rate in the plasma. The production rate (Rp) is a function of the fractional pressure of O2 (PO2), total pressure P, power W, device constant D, and reaction rate constant k.
Rp = F(PO2, P, W, D, k)
- The number of produced radicals can be considered to be the same at all pressures
- The loss rate RL is a function of the recombination rate by collisions (Rc) in the gas stage and the loss rate due to collisions on the wall
- The collision rate is a function of the three-body collision rate that follows the total pressure cubed and the loss rate to the wall, which follows the ratio of wall and total pressure to a volume of the plasma
- As the collision rate in the UV afterglow is very sensitive to pressure, PO2 /P will increase when the pressure decreases until a crossover point is achieved when the radical production rate drops below the RL and PO2 begins to drop with pressure
- The loss rate to the wall may be controlled by choosing the best place for the PRS on the vacuum chamber
- The diameter and length of the radical conductance tube control the rate of radicals that reach the chamber
According to the theory, the shorter tube provides better conductance and higher cleaning rates.
Chamber Specifics/Geometry
Generally, the saleable remote plasma cleaners available for SEMs attach the plasma radical source (PRS) for cleaning on an accessible port on the SEM chamber through KF40 or similar sized flanges. In order to attain a short connection to the chamber, the remote plasma cleaner needs to be compact and fit among other detectors, probes, GIS, and accessories connected to an SEM chamber. In case, if it takes up more angular space, the cleaning rate or functionality of other devices may be lost. In addition, the system should be compatible with turbomolecular pump vacuum systems of field-emission SEMs.
.jpg)
Figure 2. The compact Evactron E50 model fits among a forest of accessories on a crowded SEM chamber.
In order to address this problem, XEI Scientific developed the compact Evactron E50 system to plasma clean at turbo molecular pressures. The PRS employs a fixed input flow rate that is preset to eliminate the requirement for an adjustable flow valve or vacuum gauge. Since the SEM user only wants cleaning at a good rate and is not carrying out plasma studies, a vacuum gauge and flow rate adjustment are not required.
The Evactron is also compact as it incorporates an RF Hollow Cathode plasma generator, which is energy efficient. This design produces high-density plasma without extra heat generation linked with an ICP (inductively coupled plasma) coil. ICP makes use of higher power than Evactron CCP, similar to the power ratings of incandescent lamps against compact fluorescent lamps. Fluorescent lamps employ CCP technology to produce light efficiently just like the Evactron PRS employs CCP to produce Oxygen radicals efficiently. Instead of generating radicals, an ICP wastes power by heating a coil with RF current. Evactron plasma cleaners run cool, are smaller, and employ dual action UV and Plasma cleaning to offer the “Fastest Way to Pristine™”.
Materials and Methods
In order to show the effect of geometry and distance on radical loss by recombination on tube walls, tests were performed using QCMs to measure the concentration of oxygen radicals by evaluating the removal rate of a hydrocarbon film.
- An Evactron 25Z PRS was employed to produce oxygen radicals at 20 Watts RF power
- The input flow rate of air was fixed at 20 sccm
- The stainless steel radical delivery tube’s length was varied from 9 to 32 cm between the port on the chamber wall and the Evactron Zephyr™ PRS
- The PRS has shifted away from the port by adding 40 mm diameter KF 40 connectors of varying lengths between the chamber and the port
- QCMs were left in a fixed place within the 20 cm diameter chamber
- A turbopump was employed at two speeds to vary high vacuum pressure, and pumping was done only by the roughing pump at low vacuum
The experimental setup with an Evactron Zephyr PRS is shown in Figure 3. The results for different geometry and lengths of connector tube are displayed in Table 1.
.jpg)
Figure 3. Experimental set-up to show the effects of distance and radical loss on cleaning efficiency of an Evactron Zephyr PRS. (A) 9 cm straight, (B) 32 cm straight, (C) 22 cm curved connector.
Results and Discussion
Cleaning Rates versus Distance of PRS from Main Chamber
As shown in Table 1, etch rate decreases, and radical recombination increases with distance. As the distance between the PRS and the main chamber volume increases, the cleaning, and radical concentration rates decrease due to collisions with tube walls and gas species. Radicals are lost on chamber walls at pressure-dependent recombination rates in SEM vacuum chambers. When an effort is made to connect an extension pipe to shift the PRS further from the chamber due to other accessories and location of available ports, the concentration of oxygen radicals and cleaning rates decrease based on the tube length and geometry as observed in the comparison of curved vs. straight connectors.
Table 1. Turbo Plasma cleaning rates with different connector lengths and shapes. Cleaning efficiency measured at 20 watts with Zephyr system on a 22L test chamber.
Connector Type |
Straight |
Straight |
Straight |
Curved |
Straight |
PRS distance from main chamber volume (cm) |
9 |
13 |
22 |
22 |
32 |
Cleaning Rates at 20 Watts (Ang/min)* |
324 |
269 |
157 |
142 |
108 |
*Pressure during plasma operation: 2.5E-2 Torr (T-Pump cleaning mode with TMP at low speed)
Flowing UV Afterglow Cleaning
Since 2010, SEMs supplied with TMPs have grown to be standard lab equipment and the plasma cleaning could be performed at lower pressures. The cleaning efficiency and effective cleaning distance increased as a result of a longer mean free path at lower pressures. An N2 flowing UV afterglow from the plasma was noted at low pressures below 40 mTorr. The flowing UV afterglow is neutral and mostly free of ions and electrons. It includes nitrogen metastables (Figure 4) that decay in order to make the UV afterglow and oxygen radicals that oxidize the hydrocarbons. The N2+ ion causes the prominent violet peak at 384 nm. Below this are N2 metastables – the shorter UV wavelength peaks that contribute energy to surface molecules.
By desorbing water vapor as well as activating hydrocarbons for oxygen radical oxidation, afterglow UV accelerates the cleaning process. Since the flowing UV afterglow fills the entire volume of the chamber, surfaces are illuminated from all angles.
.jpg)
Figure 4. A spectrum of Evactron air plasma demonstrating characteristic UV wavelengths.
Complete Hydrocarbon Cleaning Confirmed by RGA Spectra
Spectra were taken using an Ocean spectrometer with quartz lens via a quartz window into the vacuum chamber (Figure 5A) contaminated with a known thickness of pump oil. The comparison of spectra before (Figure 5A) and after (Figure 5B) five minutes of cleaning confirms the elimination of all hydrocarbon peaks from the spectrum. The acquired results differ with various hydrocarbon contaminants.
.jpg)
Figure 5A. HC signature before cleaning.
.jpg)
Figure 5B. HC removed after 5 min cleaning.
An Example of Why Clean Samples are Critical: EBSD/TKD Data
Transmission Kikuchi diffraction methods for the scanning electron microscope, as discussed by Keller and Geiss7, can produce crystallographic data at low energies from 10 to 20 nm nanoparticles and ultrathin films. If sample surfaces are contaminated with hydrocarbons, then the spatial resolution is compromised. Plasma cleaning enhances TKD pattern quality which equals an improved effective spatial resolution, increased acquisition speed, and a better indexing rate. As illustrated in Figures 6A and 6B, after Evactron plasma cleaning, an increase of 88% in hit rate on 20 nm Au foil was noted with a Bruker e-FlashFS and OPTIMUS™ TKD8. The IPFz maps reveal a distinct enhancement of detected orientation of crystals together with a single crystallographic reference direction.
.jpg)
Figure 6A. IPFz map before plasma cleaning.
.jpg)
Figure 6B. IPFz map after plasma cleaning.
Conclusions
The latest range of Evactron remote plasma cleaners is now available for cleaning FIBs and SEMs faster and more completely compared to the first models launched in 2000. Turbomolecular pumped FIBs and SEMs can be plasma cleaned at lower pressures compared to diffusion pumped units. Operation at lower pressures allows longer distance penetration by oxygen radicals to clean larger chambers. In addition, flowing afterglow UV increases the effective cleaning area and accelerates the oxidation of hydrocarbons. As a result, higher oxygen radical concentrations can reach contaminated surfaces within all recesses of a chamber.
Smaller-sized PRS heads can be fixed closer to the chamber wall among various sensors and accessories without interference. Setting up the remote PRS close to the chamber wall port increases the cleaning rate by decreasing the recombination of oxygen radicals before they reach the main vacuum chamber. Corners and curves in the connecting tube significantly reduce oxygen radicals. If input gas flow is constant due to decreased radical recombination and increased flowing afterglow UV intensity, rates of plasma cleaning with air increase at lower pressures. Radical density and cleaning rate depends on radical production and recombination rates, chamber geometry and distances, source type and efficiency, pressure and means free path, radical flux scattering, flowing N2 afterglow, and UV intensity for triggering the surface.
References and Further Reading
- Joubert, L. M. (2013). Microscopy and Analysis, May, 15-20.
- Morgan, C. G., Gleason, M. M., Vane, R. (2007). Microsc. Microanal. 13(2), 1736.
- Gleason, M. M., Morgan, C. G. Vane, R. (2007). Microsc. Microanal. 13(2) 1734.
- Morgan, C. G., Gleason, M. M, Vane, R. (2007). Microscopy Today, 15(5), 22.
- Morgan, C. G. and R. Vane. (2012). Microsc. Microanal. 18(2), 1238.
- Vane, R. (2013). Microsc. Microanal. 19(2), 1338.
- RR Keller and RH Geiss, J. Microscopy 245(3) (2012) 245-251.
- The authors thank Dr. Daniel Goran of Bruker Nano GmbH and teams from the Technical University of Denmark DTU-Danchip company and DTU’s Center for Electron Nanoscopy in Copenhagen, Denmark for generously providing the EBSD/TKD data.
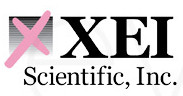
This information has been sourced, reviewed and adapted from materials provided by XEI Scientific.
For more information on this source, please visit XEI Scientific.