Gallium nitride (GaN) is a binary III/V semiconductor seen as a potential successor to silicon. GaN has a wider bandgap than silicon, meaning it can maintain higher voltages in electronic devices.1 This allows for the development of smaller, more energy-efficient components compared to those made with silicon.
GaN also excels in high-temperature environments, making it particularly valuable in applications like automotive electronics, where silicon-based technology faces design limitations.2
Beyond electronics, GaN is also extensively employed in the photonics industry as it is the material used for UV and blue light-emitting diodes (LEDs) and lasers. The discovery of this property led to the development of energy-efficient white LED lighting and earned Shuji Nakamura, Akasaki Isamu, and Amano Hiroshi being the 2014 Nobel Prize in Physics.
To produce high-quality GaN optoelectronic devices, manufacturers must minimize mechanical and electrical heterogeneity caused by strain and crystal defects. Therefore, non-destructive analytical techniques sensitive to these phenomena are essential to monitor GaN during manufacturing. These techniques include Raman and photoluminescence (PL).
In the study discussed in this article, Raman and PL characterization and imaging of a GaN device is demonstrated using an Edinburgh Instruments RMS1000 Confocal Microscope.
Both optical techniques are shown to be sensitive to the most subtle changes in the material. Coupled to a microscope, this capability is shown to deliver spatial resolution of different states within the material.

Figure 1. GaN Wurtzite crystal structure with the unit cell shaded yellow. Image Credit: Edinburgh Instruments
Materials and Methods
This study used LED chips on an adhesive film designed to emit at 520 nm as samples. The LEDs were made up of GaN-based layers grown epitaxially on sapphire and Au alloy bonding pad electrodes.
An RMS1000 was fitted with an externally coupled 325 nm He-Cd laser for PL characterization, a 638 nm laser for Raman characterization and a CCD camera in a 225 mm spectrograph, as shown in Figure 2.
A 40X 0.47 NA UV objective lens was used to probe the sample with a 325 nm laser, while a 100X 0.9 NA objective lens was employed for the 638 nm analysis. Imaging with both spectroscopic techniques was facilitated by an automated X, Y, and Z-stage, ensuring precise spatial resolution.

Figure 2. Edinburgh Instruments RMS1000 Confocal Microscope used for PL and Raman characterization of GaN. Image Credit: Edinburgh Instruments
Raman Characterization of GaN
Firstly, the GaN LED chip was studied using Raman microscopy. In the Raman spectrum shown in Figure 3, the two main bands for GaN are displayed in red. These correspond to the E2 band at 567 cm-1 and the A1 longitudinal optical (LO) phonon at 735 cm-1.3
A peak at 670 cm-1 was also seen, previously attributed to a disorder-activated vibrational mode of GaN by Cardoso et al.4 Also present were Raman bands arising due to the underlying sapphire substrate (Al2O3) at 420 cm-1 and 750 cm-1. The typical PL signature from Cr3+ doped Al2O3 was observed ca. 1300 cm-1, corresponding to about 1.786 eV (694 nm).5

Figure 3. Raman spectrum of GaN. Image Credit: Edinburgh Instruments
Spectral mapping with the 638 nm laser was used to image an area of the GaN chip. In order to determine the distribution of GaN in this area, the intensity of the E2 (high) mode was imaged, as shown in Figure 4b. The intensity was observed to be consistently higher in the region of the chip within the P- and N-GaN layers.
The position of the E2 (high) mode was mapped, as shown in Figure 4c. The Raman shift of this mode can be correlated with strain in GaN, with an increase in Raman shift relative to 567 cm-1 corresponding to compressive strain and a decrease to tensile strain.
Raman microscopy can easily visualize strain on semiconductor materials such as silicon and GaN. The yellow and red regions in Figure 4c correspond to areas where the GaN experiences compressive strain, and the black regions to areas of tensile strain.
Figure 4d shows the variation in peak position and intensity of the GaN E2 band across the images. Figure 4e shows the sapphire PL intensity, with Figure 4f displaying the corresponding spectra, demonstrating that the sapphire PL was reduced in the areas with the highest GaN Raman intensity.

Figure 4. Raman mapping of GaN. Image Credit: Edinburgh Instruments
Photoluminescence Characterization of GaN
The GaN chip was also analyzed using PL microscopy. This technique measures bandgaps in semiconductor materials directly, and due to the sensitivity of bandgap energies to impurities such as defects and dopants, microscopic analysis of devices can reveal their presence.
Figure 5 shows the PL spectrum of GaN at room temperature. It is dominated by a strong near-band-edge (NBE) emission at 3.41 eV (364 nm), correlating to the direct band-to-band recombination of electrons in the conduction band and holes in the valence band, as shown in Figure 4.6
A 325 nm He-Cd laser externally coupled to the RMS1000 was used to excite and observe this band.
PL bands at 2.33 eV (532 nm) and 1.71 eV (725 nm) were also observed. The sapphire PL band was not present in the spectrum due to the higher absorption coefficient of the surface GaN material when using the 325 nm UV excitation wavelength.

Figure 5. PL spectrum of GaN. Image Credit: Edinburgh Instruments
PL imaging was performed on the same area of the chip investigated with Raman, as shown in Figure 6. The whole region shown in Figure 6a visualized in brightfield through the UV objective was studied. False color imaging was also conducted using UV and visible bands, as shown in Figures 6b and 6c.
The 3.41 eV NBE UV PL intensity image shows that the P-GaN region of the chip does not exhibit PL. The effect of diminishing NBE PL of GaN with increasing Mg dopant concentration (P doping) has previously been reported by Hess et al.7
The N-GaN region emits UV PL strongly, with the exception of a region in the top right-hand side of the image. This is the area experiencing compressive strain, as demonstrated in the Raman image shown in Figure 4c.
The chip also emitted at 2.38 eV (520 nm), corresponding to the PL from InGaN. This is the layer sandwiched between P- and N-GaN that gives rise to the green color of the LED. The emission in this region was observed to be more intense and blue-shifted from the weak visible emissions in the N-GaN layer and was largely confined to the black strip at the boundary of the P and N layers.
Figure 6d shows the UV and green PL spectra from points 1 and 2 on both maps.

Figure 6. PL mapping of GaN. Image Credit: Edinburgh Instruments
Conclusions
The RMS1000 Raman and PL Confocal Microscope are both ideally suited to the analysis and characterization of GaN-based devices. Combining Raman and PL techniques enables the visualization of variations in crystalline structure and chemical composition across the chip.
Both techniques are extremely powerful tools for the non-destructive quality control monitoring of GaN-based devices.
Acknowledgments
Produced from materials originally authored by Matthew Berry and Stuart Thomson from Edinburgh Instruments.
References and Further Reading
- David, F. et al. (1988). Critical evaluation of the status of the areas for future research regarding the wide band gap semiconductors diamond, gallium nitride, and silicon carbide. MSEB, 1, pp.77-104.
- Kim, N.-I., et al. (2020). Piezoelectric pressure sensor based on flexible gallium nitride thin film for harsh-environment and high-temperature applications. Sensors and Actuators A: Physical, 305, p.111940. https://doi.org/10.1016/j.sna.2020.111940.
- Kuball, M. (2001). Raman spectroscopy of GaN, AlGaN and AlN for process and growth monitoring/control. 31(10), pp.987–999. https://doi.org/10.1002/sia.1134.
- Cardoso, J., et al. (2021). Exploring swift-heavy ion irradiation of InGaN/GaN multiple quantum wells for green-emitters: the use of Raman and photoluminescence to assess the irradiation effects on the optical and structural properties. Journal of Materials Chemistry C, 9(28), pp.8809–8818. https://doi.org/10.1039/d1tc01603b.
- Liu, D., et al. (2012). Al2O3:Cr3+ microfibers by hydrothermal route: Luminescence properties. Materials Research Bulletin, [online] 47(9), pp.2332–2335. https://doi.org/10.1016/j.materresbull.2012.05.026.
- Santana, G., et al. (2013). Photoluminescence Study of Gallium Nitride Thin Films Obtained by Infrared Close Space Vapor Transport. Materials, 6(3), pp.1050–1060. https://doi.org/10.3390/ma6031050.
- Hess, S., et al. (1998). Photoluminescence studies of Mg-doped and Si-doped gallium nitride epilayers. Physica Status Solidi (B) Basic Research, (online) 210(2). Available at: https://ora.ox.ac.uk/objects/uuid:e836180a-d878-45ad-abad-98c1f96ad80f (Accessed 30 Jan. 2025).
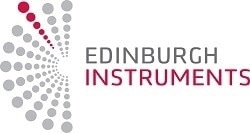
This information has been sourced, reviewed and adapted from materials provided by Edinburgh Instruments.
For more information on this source, please visit Edinburgh Instruments.