In addition to its ecologically acceptable character, Zn-ferrite (ZnFe2O4) is a spinel ferrite with a chemical element that promises relatively low manufacturing costs. Its spinel structure is relatively “open,” with numerous unoccupied crystallographic sites, allowing for the insertion of (mobile) dopants, potentially expanding the material’s range of use. This is explored further in the journal Magnetism.
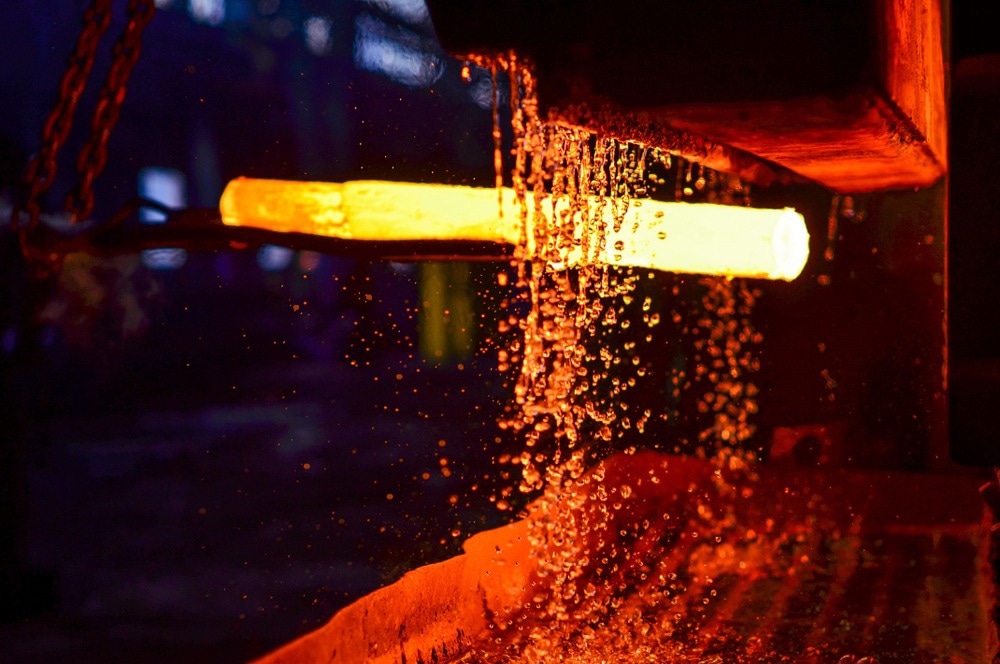
Study: Competing Magnetic Interactions in Inverted Zn-Ferrite Thin Films. Image Credit: Maksim Safaniuk/Shutterstock.com
Growth circumstances such as off-stoichiometry effects in the Zn and Fe content, nonzero Fe2+/Fe3+ ratios, and also micro/nano strains all impact the overall characteristics of nanostructured Zn-ferrite. For example, the migration of Zn cations during annealing can result in the production of two distinct crystalline phases (hematite and magnetite), as well as Zn-ferrite, which can obstruct numerous industrial uses.
In different nanostructured Zn-ferrite, ranging from nanoparticles to nanocrystalline thin films, the thermodynamics of cation disorder and the relationship between the degree of inversion and the annealing temperature has been investigated.
Even though researchers know that cation distribution (Fe3+ and Zn2+) governs the material properties of Zn-ferrite films and their emerging applications in spintronic and high-frequency devices, its low-temperature magnetic properties have not been thoroughly investigated; understanding the ordering behavior of magnetism in Zn-ferrite is critical.
Sputtering was used to create inverted Zn-ferrite thin films, which were then vacuum annealed in situ and air annealed ex situ. These films have a variety of temperature-dependent magnetic characteristics that may be described using the magnetic components model (ferrimagnetic, superparamagnetic, and paramagnetic).
Methodology
Radiofrequency (RF) magnetron sputtering of Zn-ferrite thin films (500 nm) on fused quartz substrates was carried out at an RF power of 100 and 200 W under a pure argon pressure of 1 × 10-3 mbar from a ceramic ZnFe2O4 target. X-ray diffraction (XRD) and scanning electron microscopy (SEM) was used to investigate the crystalline phase and microstructure of these films, respectively.
A physical property measuring system (PPMS) connected to a vibrating sample magnetometer was used to test the magnetic characteristics (VSM). Temperature dependence of magnetization (M-T) data was collected in a comparatively large applied field of 1.59 × 106 A/m under cooled circumstances ranging from 300 to 5 K. The magnetization data of films were removed from the diamagnetic contribution of the quartz substrate.
Results
Figure 1 shows the XRD patterns of as-grown Zn-ferrite thin films as well as those annealed in the air (ex situ) and vacuum (in situ), demonstrating the creation of a single-phase FCC cubic spinel Zn-ferrite structure.
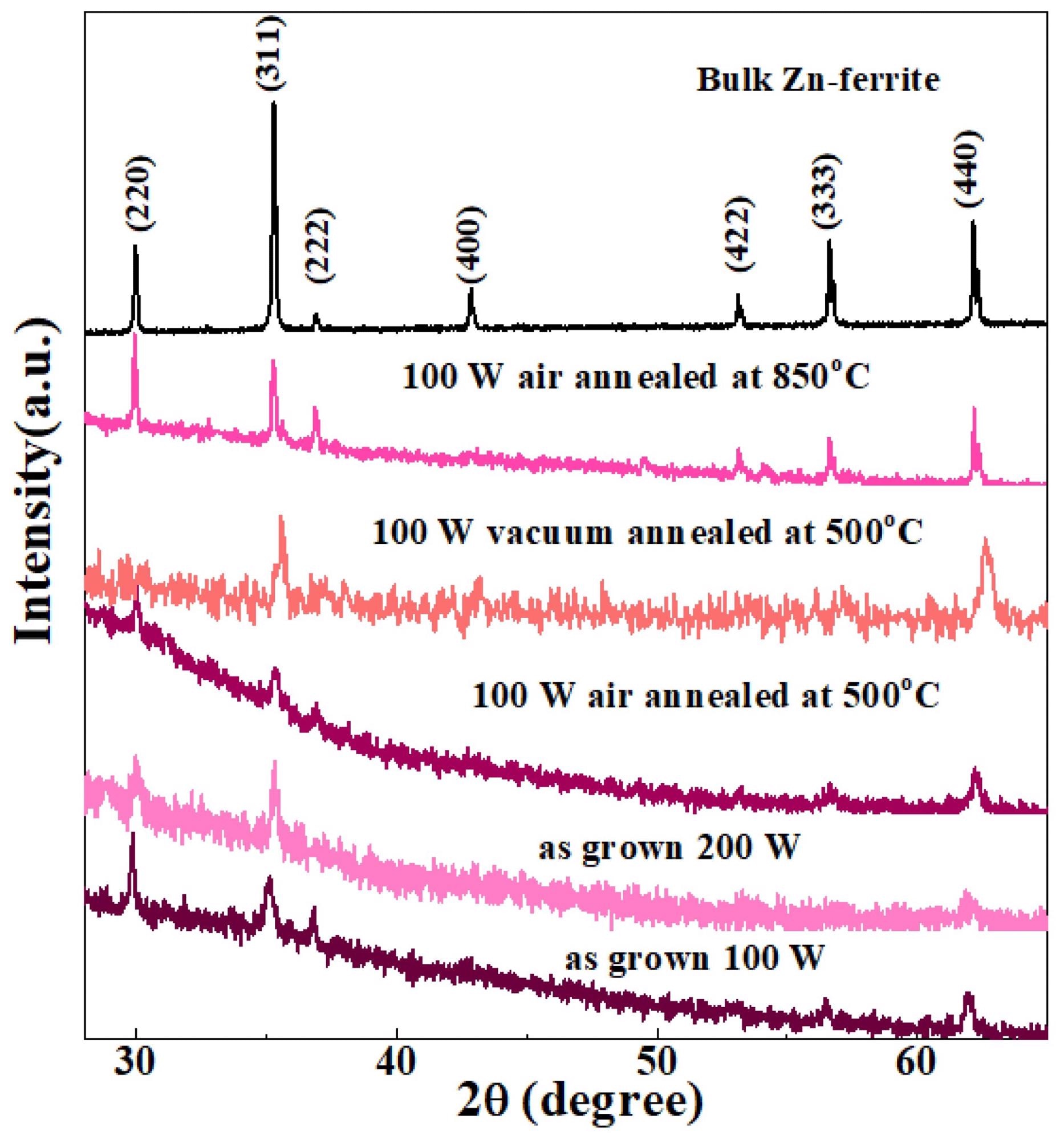
Figure 1. XRD patterns of as-grown, air-annealed, and vacuum-annealed Zn-ferrite thin films along with bulk Zn-ferrite data. Image Credit: Bohra, et al., 2022
Table 1 shows the lattice constants for these films, which vary from 8.41–8.46 Å, compared to the bulk Zn-ferrite value of 8.44 Å.
Table 1. Lattice constants, grain sizes, and M-T data fitting parameters. Source: Bohra, et al., 2022
Sample |
Lattice Constant
(Å) |
Grain Size
(nm) |
β |
TC or θ
(K) |
α |
as-grown 100 W |
8.46 |
24 |
1.25 |
604 |
0.85 |
as-grown 200 W films |
8.43 |
30 |
1.25 |
600 |
0.8 |
100 W films air annealed at 500 °C |
8.43 |
31 |
1.45 |
452 |
1 |
100 W films air annealed at 850 °C |
8.44 |
58 |
- |
254 |
0 |
100 W films vacuum annealed at 500 °C |
8.41 |
41 |
2.5 |
828 |
1 |
The Scherrer formula (Table 1) estimates that grain sizes for as-grown films are in the range of 24–30 nm and rise (31–58 nm) during annealing (regardless of the environment), which is verified by SEM pictures (see Figure 2).
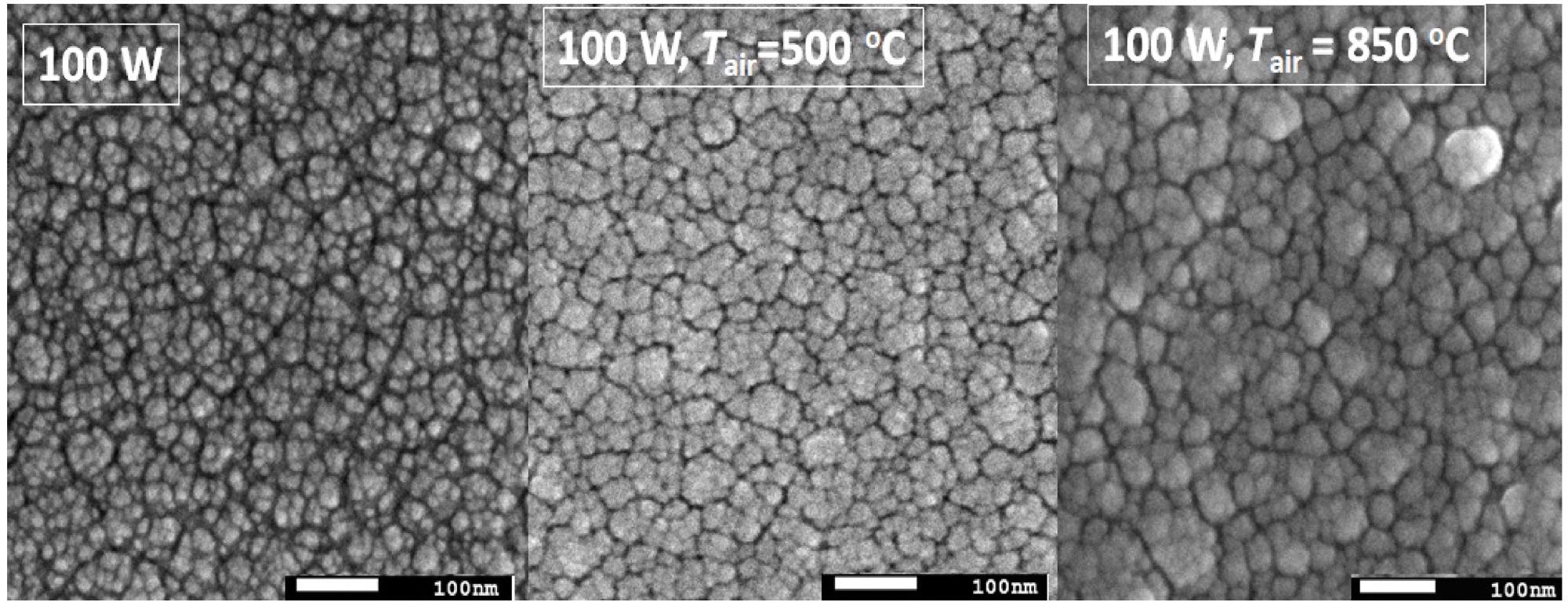
Figure 2. SEM images of as-grown Zn-ferrite thin films (deposited at an RF power of 100 W), which were later annealed at 500 °C and 850 °C in the air. Image Credit: Bohra, et al., 2022
First, the impact of ex situ air annealed on the temperature dependence of magnetism of Zn-ferrite thin films was studied. Figure 3 shows the field-cooled (FC) magnetizations of as-grown and air-annealed samples.
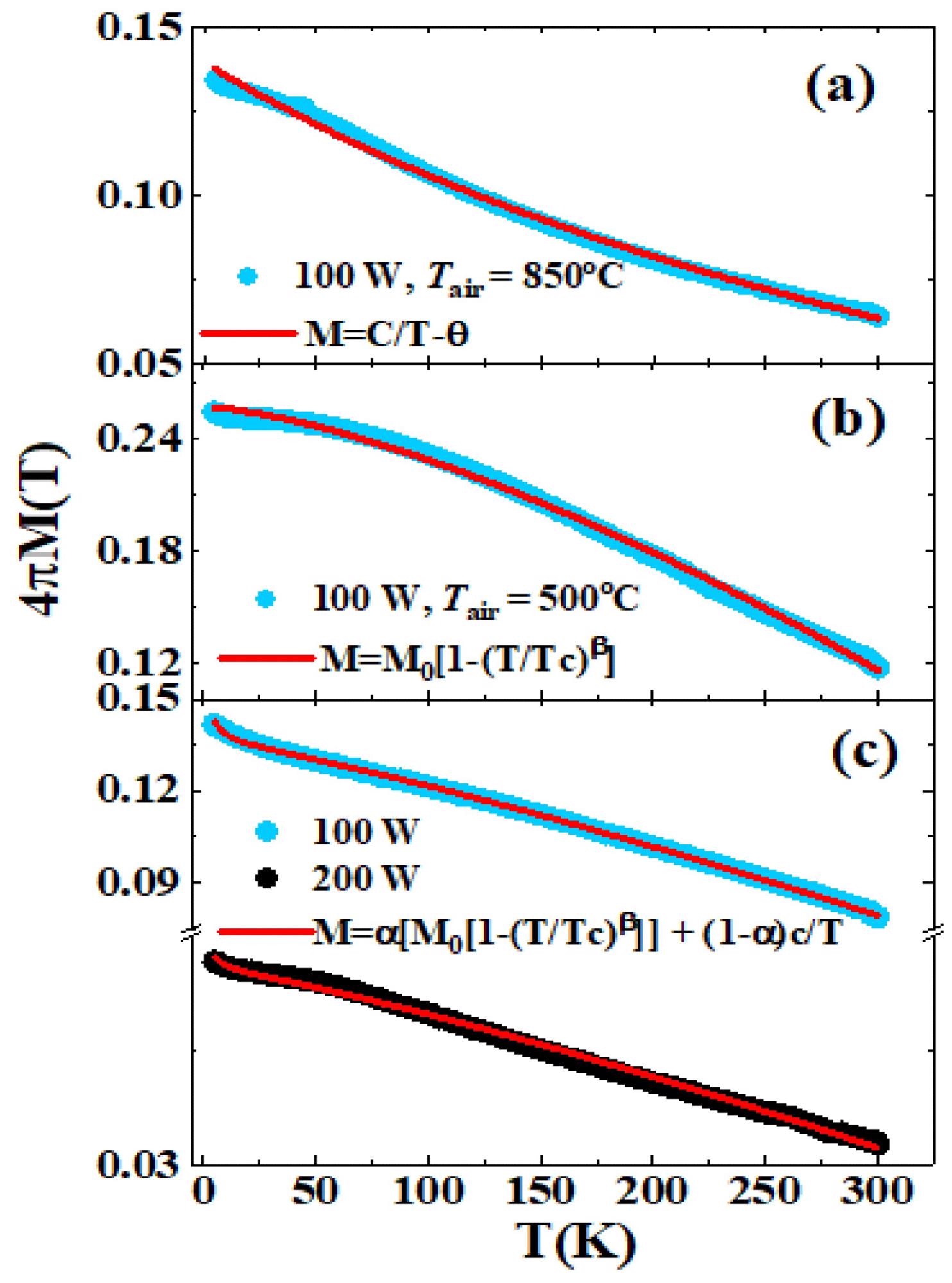
Figure 3. FC M-T curves measured at the fixed field of 1.59 × 106 A/m for as-grown (a) and air-annealed Zn-ferrite thin films (b, c). Red lines indicate fitted data with a component model. Image Credit: Bohra, et al., 2022
The field dependence of the magnetization (M–) curve was evaluated at a low temperature to evaluate the above unique magnetic behaviors found in the Zn-ferrite thin films, shown in Figure 4 for both as-grown and air-annealed samples.
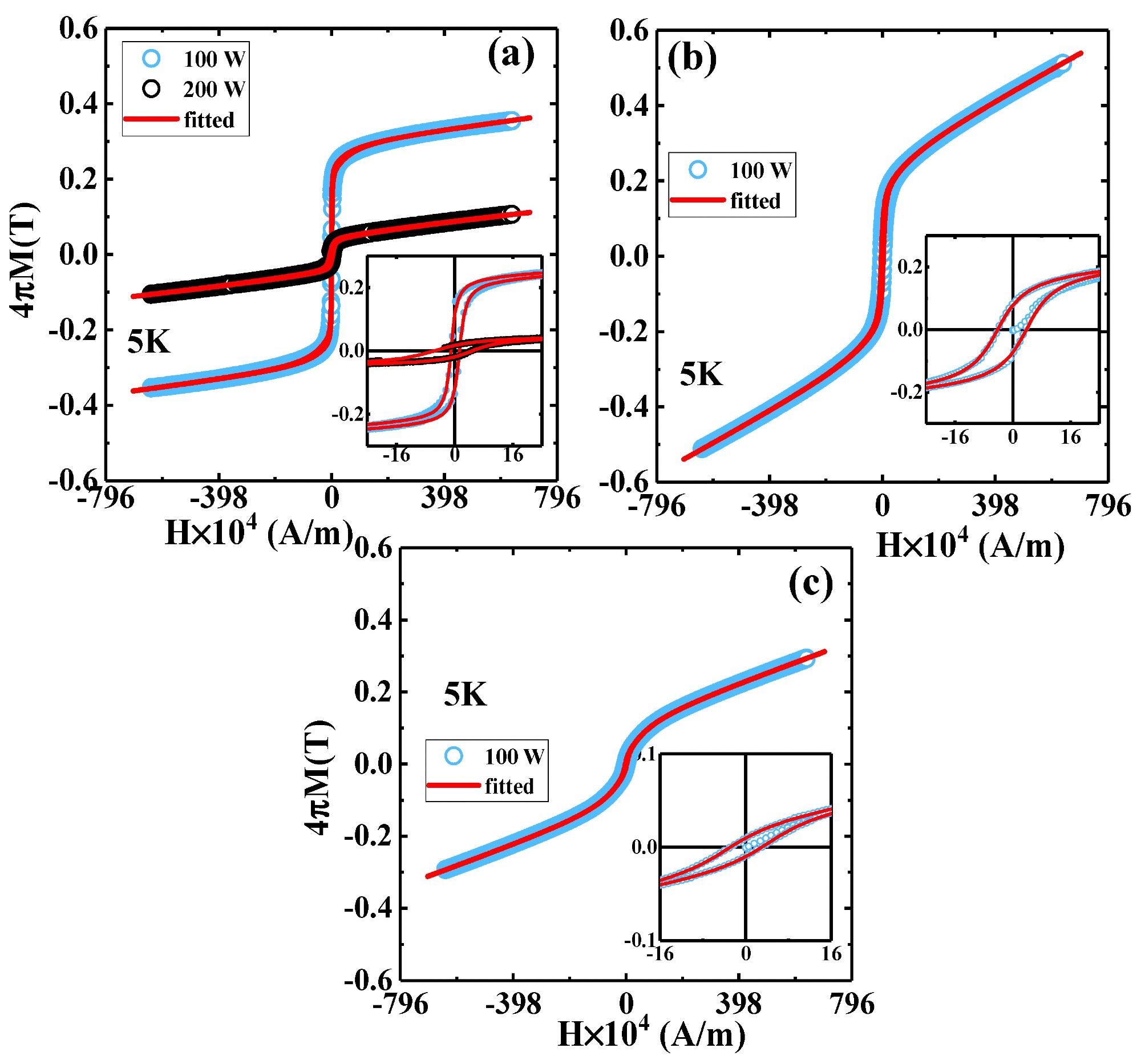
Figure 4. M−H loops (open symbols) for as-grown films (a) and films annealed at 500 °C (b) and 850 °C (c) along with fitted curves (solid lines). The insets show the low-field regions of M–H curves. Image Credit: Bohra, et al., 2022
Table 2 lists all of the extracted fitting parameters. As-grown films formed at 100 and 200 W have the highest overall FM contributions, but the PM or AFM contribution increases from ~11 to ~17% as the annealing temperature rises.
Table 2. Squareness ratio: S = Mr/MS, saturation magnetization: MS, magnetic susceptibility: χ, coercivity: HC, and α1 and α2. Source: Bohra, et al., 2022
Sample |
HC1
(kA/m) |
HC2
(kA/m) |
MSf1
(T) |
MSf2
(T) |
S1 |
S2 |
α1 |
α2 |
1 - α1 - α2 |
Xf |
as-grown 100 W |
9.55 |
92.95 |
0.35 |
0.19 |
0.34 |
0.25 |
0.597 |
0.402 |
0.001 |
473 |
as-grown 200 W |
45.67 |
446.111 |
0.048 |
0.02 |
0.20 |
0.13 |
0.984 |
0.001 |
0.016 |
0.47 |
100 W air annealed at 500 °C |
38.28 |
42.73 |
0.39 |
0.21 |
0.31 |
0.17 |
0.251 |
0.636 |
0.112 |
0.32 |
100 W air annealed at 850 °C |
14.40 |
36.37 |
0.16 |
0.13 |
0.06 |
0.03 |
0.105 |
0.722 |
0.173 |
0.13 |
100 W vacuum annealed at 500 °C |
35.5 |
32.06 |
0.063 |
0.16 |
0.15 |
0.41 |
0.452 |
0.52 |
0.003 |
0.12 |
Figure 5a illustrates the FC magnetization behavior of in situ vacuum-annealed (500 °C) Zn-ferrite films; it reveals an anomaly below 130 K, which differs from conventional ferrimagnetic behavior as shown by the red dashed line. These films’ M–H loops (see Figure 5b) have decreased saturation magnetization MS values (Table 2), indicating that the valence state of Fe has changed.
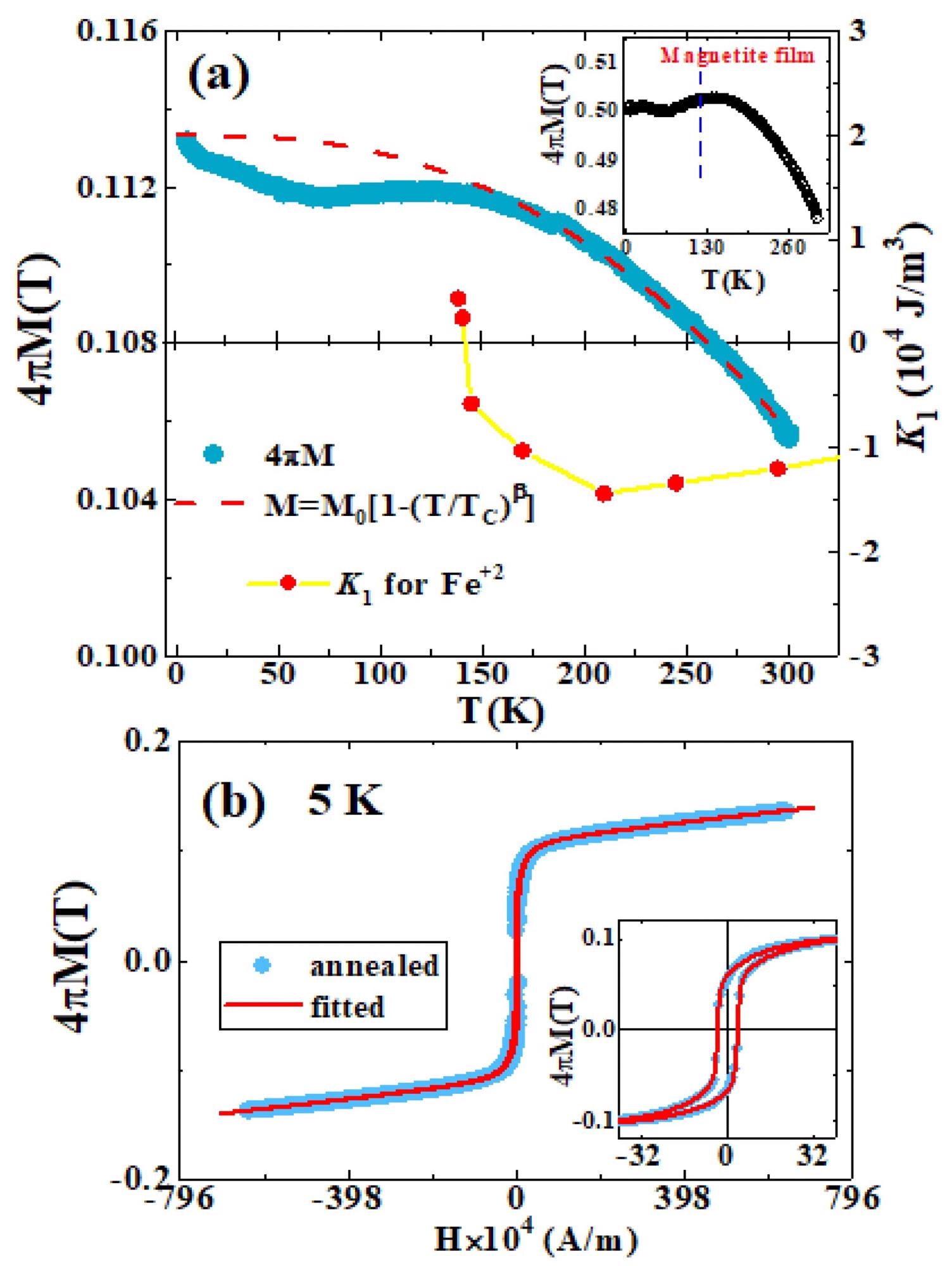
Figure 5. The M-T curve measured at 1.59 × 106 A/m for vacuum-annealed Zn-ferrite films (a). Red dashed lines indicate fitted data to the Bloch law. The red color closed symbol data for the K1 anisotropy constant of magnetite was taken from reference. The inset shows the M-T curve of magnetite thin films. The M−H loops of vacuum-annealed Zn-ferrite films were taken at 5 K (b). Image Credit: Bohra, et al., 2022
This cation inversion technique differs from both the ex situ air-annealed Zn-ferrite films and the in situ air-annealed Zn-ferrite films [Zn1-x+2Fex+3]A[Znx+2Fe2-x+3]BO4 with the same annealing temperatures as ZnxFe3xO4 (see Table 2): [Znx+2Fe1−x+3]A[Fe1+x+3Fe1−x+2]BO4 (see Figure 6), in which magnetization values rise as Zn concentration falls during heat treatment, especially in a reducing environment.
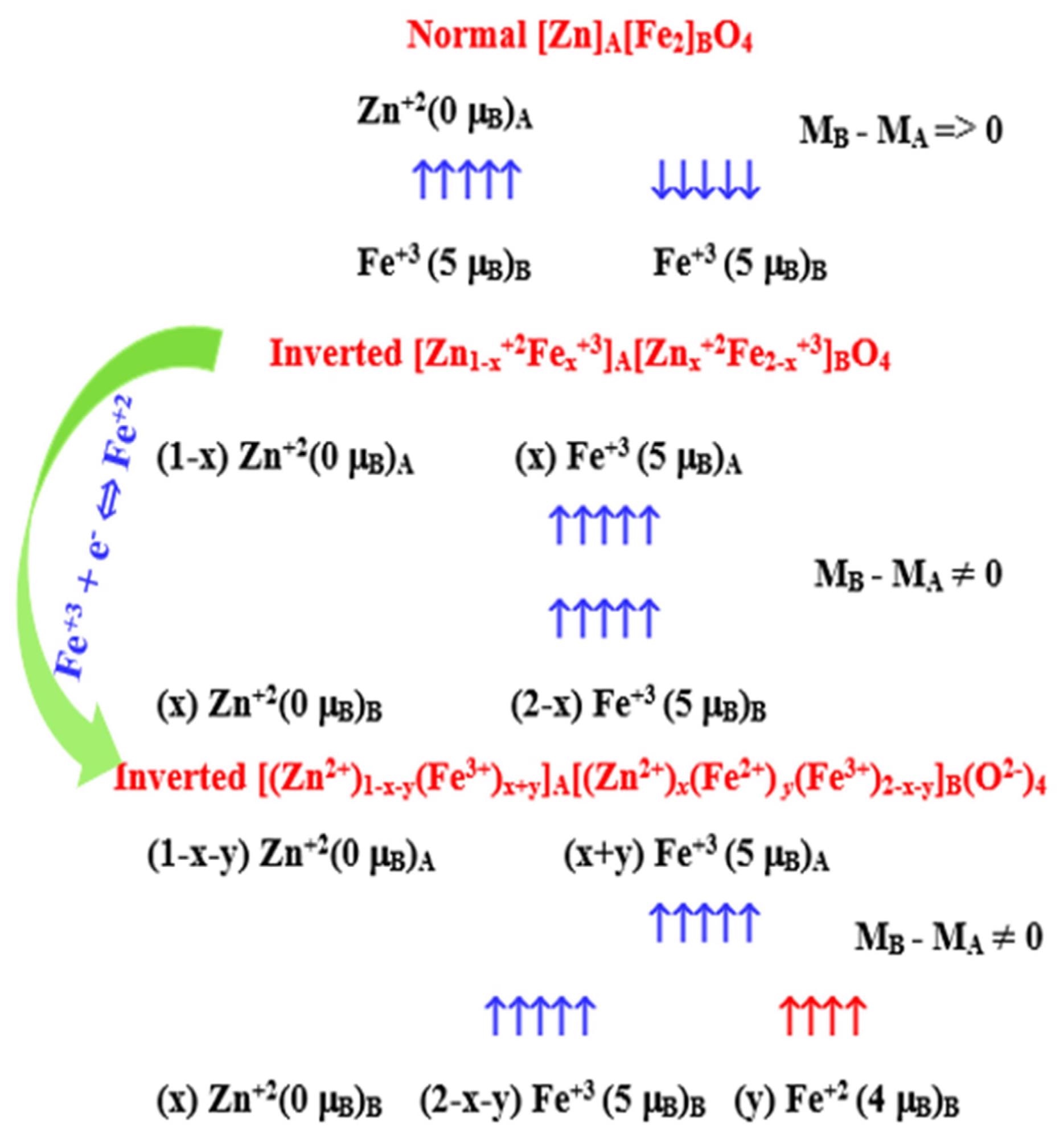
Figure 6. Various spin arrangement schemes in ZnFe2O4. Image Credit: Bohra, et al., 2022
Researchers also sputtered magnetite films from the hematite (Fe2O3) target under comparable growth and annealing circumstances as Zn-ferrite films to further validate that the observed anomaly is due to the shift in the valence state of Fe, that is, the presence of Fe+2 ions as a controlled experiment.
Scientists discovered an analog of the magnetization decline below 130 K in FC magnetization curves of magnetite films (see inset of Figure 5a), but with larger net magnetization values across the temperature range.
As a result of this research, it is concluded that the magnetic properties of nanocrystalline Zn-ferrite films are extremely sensitive to not only growth conditions but also post-annealing treatments and their environment, resulting in a variety of grain sizes, Fe2+/Fe3+ ratios, and magnetic interactions.
Conclusion
The goal of this study was to understand the underlying magnetism of nanocrystalline Zn-ferrite thin films, which is an antiferromagnet in its bulk form. On these films, multiple types of in situ and ex situ annealing processes were applied, each causing cation inversion in the Zn-ferrite spinel structures in a distinct way. The following are the primary findings that can be derived from this research.
Researchers effectively described the temperature- and field-dependent magnetic characteristics of sputtered Zn-ferrite thin films using the component (SPM, FM, and PM) model, suggesting that the model may be the most suited for multicomponent magnetic systems.
With increasing annealing temperature, ex situ air annealing transforms the combined dominance of the SPM and FM contribution to FM and, finally, bulk-type AFM state while keeping the single magnetic ion (Fe+3) character.
On the other hand, in situ vacuum annealing causes a partial transition of octahedral Fe+3 ions into Fe+2 ions, reducing the saturation magnetization value and generating an abnormality in low-temperature magnetization data at about 130 K.
Finally, the adjustable magnetic properties of sputtered Zn-ferrite thin films provide possibilities in spintronics and high-frequency devices due to their low processing temperature and ease of integration with semiconductor technology.
Journal Reference:
Bohra, M., Battula, S.V., Singh, N., Sahu, B., Annadi, A. and Singh, V. (2022) Competing Magnetic Interactions in Inverted Zn-Ferrite Thin Films. Magnetism, 2(2), pp.168-178. Available Online: https://www.mdpi.com/2673-8724/2/2/12/htm
References and Further Reading
- Bohra, M., et al. (2021) Nanostructured ZnFe2O4: An Exotic Energy Material. Nanomaterials, 11, p. 1286. doi.org/10.3390/nano11051286.
- Fritsch, D (2018) Electronic and optical properties of spinel zinc ferrite: Ab initio hybrid functional calculations. Journal of Physics: Condensed Matter, 30, p. 095502.
- Heda, N. L., et al. (2020) Performance of hybrid functional in linear combination of atomic orbitals scheme in predicting electronic response in spinel ferrites ZnFe2O4 and CdFe2O4. Journal of Materials Science, 55, pp. 3912–3925. doi.org/10.1007/s10853-019-04289-8.
- Ulpe, A. C., et al. (2019) Influence of spin state and cation distribution on stability and electronic properties of ternary transition-metal oxides. ACS Omega, 4, pp. 4138–4146. doi.org/10.1021/acsomega.8b03254.
- Kim, J. H., et al. (2020) Ferrites: Emerging light absorbers for solar water splitting. Journal of Materials Chemistry A, 8, pp. 9447–9482. doi.org/10.1039/D0TA01554G.
- Harris, V G (2012) Modern Microwave Ferrites. IEEE Transactions on Magnetics, 48, pp. 1075–1104. doi.org/10.1109/TMAG.2011.2180732.
- Bohra, M., et al. (2006) Large room temperature magnetization in nanocrystalline zinc ferrite thin films. Applied Physics Letters, 88, p. 262506. doi.org/10.1063/1.2217253.
- Zviagin, V., et al. (2020) Control of magnetic properties in spinel ZnFe2O4 thin films through intrinsic defect manipulation. Journal of Applied Physics, 128, p. 165702. doi.org/10.1063/5.0019712.
- Marcu, A., et al. (2007) Transport properties of ZnFe2O4−δ thin films. Journal of Applied Physics, 102, p. 023713. doi.org/10.1063/1.2751492.
- Granone, L. I., et al. (2018) Effect of the degree of inversion on optical properties of spinel ZnFe2O4. Physical Chemistry Chemical Physics, 20, pp. 28267–28278. doi.org/10.1039/C8CP05061A.
- Nakashima, S., et al. (2005) High magnetization and the high-temperature superparamagnetic transition with intercluster interaction in disordered zinc ferrite thin film. Journal of Physics: Condensed Matter, 17, pp. 137–149.
- Jedrecy, N., et al. (2014) Magnetic and magnetotransport properties of ZnxFe3−xO4−y thin films. Journal of Applied Physics, 116, p. 213903. doi.org/10.1063/1.4903211.
- Rivero, M., et al. (2016) Synthesis and structural characterization of ZnxFe3−xO4 ferrite nanoparticles obtained by an electrochemical method. RSC Advances, 6, pp. 40067–40076. doi.org/10.1039/C6RA04145K.
- Yu, G., et al. (2015) Selective reduction process of zinc ferrite and its application in treatment of zinc leaching residues. Transactions of Nonferrous Metals Society of China, 25, pp. 2744–2752. doi.org/10.1016/S1003-6326(15)63899-7.
- Cobos, M.A., et al. (2019) Magnetic phase diagram of nanostructured zinc ferrite as a function of inversion degree δ. The Journal of Physical Chemistry C, 123, pp. 17472–17482. doi.org/10.1021/acs.jpcc.9b02180.
- Singh, J.P., et al. (2022) Effect of thermal annealing on the film and substrate/film interface: The case of ZnFe2O4. Applied Nanoscience, doi.org/10.1007/s13204-021-02129-3.
- Lin, W. J., et al. (2013) Exchange bias and magneto-resistance in an all-oxide spin valve with multi-ferroic BiFeO3 as the pinning layer. Acta Materialia, 61, pp. 7444–7453. doi.org/10.1016/j.actamat.2013.08.054.
- Sai, R., et al. (2015) Oriented nanometric aggregates of partially inverted zinc ferrite: One-step processing and tunable high-frequency magnetic properties. Journal of Applied Physics, 117, p. 17E511. doi.org/10.1063/1.4916816.
- Sai, R., et al. (2017) Magnetic nanoferrites for RF CMOS: Enabling 5G and beyond. The Electrochemical Society Interface, 26, pp. 71–76.
- Bohra, M., et al. (2011) Narrow ferromagnetic resonance line width polycrystalline Zn-ferrite thin films. IEEE Transaction on Magnetics, 47, pp. 345–348. doi.org/10.1109/TMAG.2010.2081664.
- Monsalve, J. G., et al. (2021) Insight into magnetic properties in zinc ferrite thin films by tuning oxygen content. Current Applied Physics, 22, pp. 77–83. doi.org/10.1016/j.cap.2020.12.015.
- Bohra, M., et al. (2013) Low temperature magnetization studies of nanocrystalline Zn-ferrite thin films. IEEE Transaction on Magnetics, 49, pp. 4249–4252. doi.org/10.1109/TMAG.2013.2239969.
- Nakashima, S., et al. (2007) Thermal annealing effect on magnetism and cation distribution in disordered ZnFe2O4 thin films deposited on glass substrates. Journal of Magnetism and Magnetic Materials, 310, pp. 2543–2545. doi.org/10.1016/j.jmmm.2006.11.144.
- Dash, J., et al. (1999) Study of magnetization and crystallization in sputter deposited LiZn ferrite thin films. Journal of Applied Physics, 86, pp. 3303–3311. doi.org/10.1063/1.371206.
- Srivastava, C. M., et al. (1976) Magnetic ordering and domain-wall relaxation in zinc-ferrous ferrites. Physical Review B, 14, pp. 2032–2040. doi.org/10.1103/PhysRevB.14.2032.
- Bohra, M., et al. (2011) Role of Ru vacancies in the magnetism of strain relaxed SrRuO3 films on SrTiO3 substrates. Journal of Applied Physics, 109, p. 07D728. doi.org/10.1063/1.3561768.
- Bohra, M., et al. (2016) Characterizing the phase purity of nanocrystalline Fe3O4 thin films using Verwey transition. Journal of Magnetism and Magnetic Materials, 418, pp. 137–142. doi.org/10.1016/j.jmmm.2016.02.010.
- Kamazawa, K., et al. (2003) Magnetic neutron scattering measurements on a single crystal of frustrated ZnFe2O4. Physical Review B, 68, 024412. doi.org/10.1103/PhysRevB.68.024412.
- Bohra, M., et al. (2021) Design of various Ni–Cr nanostructures and deducing their magnetic anisotropy. Applied Nanoscience, doi.org/10.1007/s13204-021-01998-y.
- Saha, R., et al. (2009) Evolution of ferromagnetic like order in Fe2V1−xCrxAl Heusler alloys. Physical Review B, 79, p. 174423. doi.org/10.1103/PhysRevB.79.174423.
- Philip, J., et al. (2007) Effect of thermal annealing under vacuum on the crystal structure, size, and magnetic properties ZnFe2O4 nanoparticles. Journal of Applied Physics, 102, p. 054305. doi.org/10.1063/1.2777168.
- Bohra, M., et al. (2022) Multiple spintronic functionalities into single zinc-ferrous ferrite thin films. Journal of Alloys and Compounds, 895, p. 162425. doi.org/10.1016/j.jallcom.2021.162425.
- Bickford, L. R & Jr. (1953) The Low Temperature Transformation in Ferrites. Reviews of Modern Physics, 25, p. 75. doi.org/10.1103/RevModPhys.25.75.
- Ozdemir, O & Dunlop, D J (1999) Low-temperature properties of a single crystal of magnetite oriented along principal magnetic axes. Earth and Planetary Science Letters, 165, pp. 229–239. doi.org/10.1016/S0012-821X(98)00269-6.
- Ozdemir, O (2000) Coercive force of single crystals of magnetite at low temperatures. Geophysical Journal International, 141, pp. 351–356. doi.org/10.1046/j.1365-246x.2000.00081.x.
- Muxworthy, A R & McClelland, E (2000) Review of the low-temperature magnetic properties of magnetite from a rock magnetic perspective. Geophysical Journal International, 140, pp. 101–114. doi.org/10.1046/j.1365-246x.2000.00999.x.
- Bohra, M., et al. (2019) A Short Review on Verwey Transition in Nanostructured Fe3O4 Materials. Journal of Nanomaterials, p. 8457383. doi.org/10.1155/2019/8457383.
- Zhang, H., et al. (2022) Room-temperature magnetoresistive and magnetocaloric effect in La1−xBaxMnO3 compounds: Role of Griffiths phase with ferromagnetic metal cluster above Curie temperature. Journal of Applied Physics, 131, p. 043901. doi.org/10.1063/5.0078188.
- Wang, H., et al. (2021) Spin glass feature and exchange bias effect in metallic Pt/antiferromagnetic LaMnO3 heterostructure. Journal of Physics: Condensed Matter, 33, p. 285802. doi.org/10.1088/1361-648X/ac0023
- Wang, H., et al. (2014) Three-dimensional strain state and spacer thickness-dependent properties of epitaxial Pr0.7Sr0.3MnO3/La0.5Ca0.5MnO3/Pr0.7Sr0.3MnO3 trilayer structure. Journal of Applied Physics, 115, p. 233911. doi.org/10.1063/1.4884995.