During one-dimensional gas chromatographic (1D-GC) analyses of complex biological, environmental, or petrochemical samples, the outcome is often a chromatogram that has a huge portion of unresolved components. Although it is possible to use mass spectrometry to resolve part of the complexity, large concentration differences and structural isomers can complicate the spectral interpretation and data analysis.
A narrow-bore, long, efficient, thin-film capillary column can be used to improve some chromatographic resolution, but decrease in sample loading capacity and increase in analysis time may be unappealing for high-throughput laboratories with complex samples.
In multidimensional gas chromatography (MDGC or 2D-GC), two separate columns with two distinctive stationary phases are used, thereby increasing resolution. Heart-cutting is one type of MDGC. Following an initial assessment of the sample, a part of the unresolved GC effluent can be diverted to a different column before detection. Despite the fact that heart-cutting could be a simple method for realizing a better separation of a complex mixture, it is possible to improve only a part of the one-dimensional separation using the second-dimension column.
Comprehensive two-dimensional gas chromatography (GCxGC) involves the use of a high-frequency modulator to divert the whole amount of one-dimensional effluent onto a second-dimension column. Due to the fact that every peak is transferred to the second-dimension column, prior knowledge of the separation (or lack thereof) is not needed to optimize the analysis. The first and the second dimension retention times are recorded by the detector, and a chromatographic plane (or contour plot) (Figure 1) is created by plotting the response.
When compared to a traditional 1D-GC analysis, the chromatographic separation space is drastically increased, thereby achieving a huge increase in potential peak capacity. Apart from increased resolution, the resulting chromatogram is also structured, thus making similar compounds to elute in clustered bands over the chromatogram plane.
The clustering of structurally and chemically similar compounds serves as a beneficial pointer that can help in peak identification. Increase in compound detectability is another positive outcome of a GCxGC analysis. Upon quickly trapping and “injecting” the effluent of the primary column, the modulator focuses the peaks only a few seconds prior to being detected, thereby improving the signal-to-noise ratio (S/N).
.jpg)
Figure 1. Overview of GCxGC: Peaks eluting from the 1st dimension column enter the 2nd dimension column through the modulator. The modulator rapidly traps the effluent from the primary column and “injects” this effluent to the secondary column. A fast detector simultaneously records the 1st dimension retention time and 2nd dimension retention time. A linear chromatogram is used for quantitation (e.g. integration, peak area, peak height, S/N), and the contour plot is a topographic type surface that is useful for data review and display purposes.
More time may be needed before novel advancements in technology such as GCxGC can be adopted widely. For instance, the transformation of usual techniques from packed GC to capillary GC took several decades (one could even argue that the transfer is still ongoing!). It was in 1957 that the improved resolution of a capillary GC analysis was first demonstrated with the separation of m- and p-xylene [1].
The early adopters of capillary GC were petroleum chemists, who made the most of the increased peak capacity (or resolution) of complex samples. The method was superior to packed columns, but large-scale utilization was gradual due to the robust nature and ease of use of the capillary column (glass).
In 1979, the robustness challenge was finally overcome with the introduction of fused silica capillary columns [2]; however, it has still taken several decades to transform routine established techniques from packed to capillary column technology. Capillary columns have indisputable advantages and have advanced our understanding of the chemical composition of innumerable samples.
Quite similar to the transformation of technology from packed columns to capillary columns, the transfer of 1D-GC to GCxGC in regulated industries has been gradual. The method was first demonstrated in 1991 [3]. Early adopters discovered that the increased peak capacity was helpful in the case of complex petrochemical and environmental samples.
In 2002, the commercialization of a GCxGC instrument fitted with a thermal modulator [4] initiated studies of a large cross-section of application areas (for example, environmental, petrochemical, food safety, metabolomics, fragrance). Similar challenges of robustness and ease of use have restricted the adoption of the GCxGC platform.
It has been considered that development and optimization of the method are challenging and tedious, and can be achieved only by an experienced analyst. Recently, these challenges have been reduced, rendering it easy to be implemented into a routine laboratory. Assistive guidelines [5] and tools [6] have been created to help users in developing the GCxGC method. Commercialization of flow modulators has been carried out for users looking forward to using GCxGC at a lower initial cost. Robustness of the method has been demonstrated with various published validated methods [7–11].
At present, GCxGC is in the process of being transferred from academic promotion to day-to-day practical analysis. In a routine laboratory, a comprehensive two-dimensional gas chromatographic analysis can save time by blending various compound categories into a single analysis, and simplify sample preparation and cleanup by chromatographically resolving matrix interferences. In case comprehensive characterization of a sample is required, a GCxGC analysis will speed up the discovery and offer insights into a sample that no other chromatographic method can match. More low-cost detectors, such as the FID and ECD, can be adopted for routine analyses rather than depending on mass spectrometers to resolve complex mixtures.
Save Time with Multi-Class Analyses
In routine high-throughput laboratories, saving time relates to making more money. For instance, laboratories assigned with the task of monitoring environmental pollutants are facing dirty samples (for example, sediments, soils, sludge), decreased operating budgets, and an ever-increasing cocktail of chemical pollutants. Usually, contaminated samples contain various classes of components. Contaminants such as brominated flame retardants (BFRs) and polychlorinated biphenyls (PCBs) are both halogenated compounds that are, in general, analyzed with the help of an ECD.
Since the ECD is a non-specific detector, it is necessary to carefully fractionate the sample extracts to prevent interferences. Implementation of a GCxGC technique can reduce or completely eliminate the fractionation steps. It would be possible to analyze several classes of components in a single analysis by taking advantage of the second-dimension separation space (Figure 2).
.jpg)
Figure 2. Multiple halogenated contaminants can be analyzed in a single injection using GCxGC-µECD. Brominated flame retardants (BFRs) and polychlorinated biphenyls (PCBs) are often found in the same sample extract. GCxGC reduces the need for fractionation steps.
The Canadian Ministry of the Environment and Climate Change (MOECC) Laboratory Services Branch (Toronto, Ontario) routinely uses three validated GCxGC-µECD techniques [12–14]. Not only are the GCxGC techniques validated but they also take part in proficiency testing programs offered by an external organization to fulfill accreditation requirements. Two of these techniques have been successfully accredited. In 2011, the first regulatory GCxGC technique was devised at the MOECC for a single analysis of organochlorine pesticides (OCPs), PCBs, and chlorobenzenes (CBz) in sediments, soils, and sludge through GCxGC-µECD [8].
The micro-electron capture detector (µECD) is best suited for an environmental monitoring laboratory due to its superior sensitivity for halogenated compounds, low dead volume, and low operating cost. Using GCxGC-µECD, nearly six injections were replaced with a single analysis that targeted 118 compounds. Apart from reducing analysis time, there was also a reduction in the sample preparation time since fractionation of the extracts was not required any longer. The performance of the technique was assessed using certified reference materials and exhibited exceptional agreement with certified values.
Aroclor calculations could still be made using the targeted 82 PCB congeners, thereby enabling historical comparisons to be made. Another significant advantage of the GCxGC-µECD analysis is its potential to detect non-targeted halogenated contaminants that may have been hidden in a one-dimensional analysis.
The locations of the obscure compounds in the GCxGC chromatogram can help in tentative identification or compound-specific classification (for example, dioxins, PBDEs). The potential to screen for non-targeted analytes that could detect emerging contaminants, or to capture illegal activity that would otherwise go unobserved, could be beneficial for monitoring programs.
Reduce Solvent Usage with Simplified Sample Preparation
In the case of pesticide residue analysis in food and agricultural samples, sample preparation time can be lengthy. The QuEChERS — or Quick, Easy, Cheap, Effective, Rugged, and Safe — sample preparation technique is a multi-residue general extraction and cleanup technique that saves time per sample in high-throughput food-safety laboratories. The broad-based extraction strategy is beneficial for a concurrent multi-class residue analysis, but co-extractive matrix components that exist in the extract can interfere with targeted analytes. Interferences from pesticides of interest can be chromatographically eliminated and the trouble of extensive cleanup procedures can be reduced by using the second-dimension separation space in a GCxGC analysis (Figure 3).
.jpg)
Figure 3. GCxGC-TOFMS was used to analyze a QuEChERS extract of finished tobacco. In a 1D-GC analysis matrix interference would have obscured the detection of a widely used pesticide ingredient, piperonyl butoxide. The automated peak find in the LECO ChromaTOF software identified piperonyl butoxide, with a library similarity of 876.
The QuEChERS sample preparation technique has been customized for applications other than pesticide residues in vegetables and fruit. By modifying the extraction solvent, the QuEChERS technique can be used to determine BFRs, PCBs, and polycyclic aromatic hydrocarbons (PAHs) in high-fat animal-feed ingredients (for example, fish oil, palm oil, rapeseed oil) [11]. A cleanup step is mandatory before injection on the gas chromatograph to eliminate the non-volatile lipids that exist in the extract. The co-extractive lipids are usually removed by using gel permeation chromatography (GPC), but it is time and solvent intensive.
The majority of fat can be eliminated before the analysis by performing a simple cleanup using silica solid phase extraction (SPE) columns. The SPE cleanup may not synthesize highly clean extracts as GPC, but a GCxGC analysis can chromatographically resolve co-extractives that would interfere with a 1D-GC analysis.
Such a simplified sample preparation technique (QuEChERS extraction with silica SPE cleanup) was adopted in a validated screening technique for emerging BFRs, PCBs, PAHs, and PBDEs in animal feed and ingredients using GCxGC with a time-of-flight mass spectrometer (GCxGC-TOFMS) for analysis [11].
For each compound class, various marker compounds were selected and were detected at or below the established maximum regulatory limit (MRL). The validation procedure determined with higher certainty (95%) that the screening technique would accurately flag non-compliant samples for further confirmation. Data processing was automated to rapidly identify other potential chlorinated or brominated contaminants for further expansion of the application of the screening technique. The extensive analysis of GCxGC-TOFMS also offers a comprehensive record of a sample that can be used for retrospective data mining.
Decrease Manual Labor with Automated Peak Identification
With the increase in the number of prospective chemical contaminants (in the people, environment, animals, food, etc.), there is an increased need for routine non-target screening techniques. One of the advantages of adopting GCxGC is the structured elution of components in a two-dimensional contour plot. With the help of a non-specific detector, such as the ECD or FID, the contour plot can be used to group compounds depending on their chemical class. A mass spectrometer (MS) can be used as a detector to further identify unknown peaks by searching a mass spectral library (for example, NIST).
Library matching in a 1D-GC analysis can be complex when a number of co-elutions interfere with the mass spectrum, thereby leading to search results of poor quality. The quality of the search is dependent on the conformity of the selected spectrum to the reference mass spectrum.
A GCxGC-TOFMS can help in unknown peak identification in a few ways. First, the extra peak capacity in GCxGC relates to more resolved peaks. Better resolving a peak from surrounding interferences will likely result in a high library match (so long as the analyte in question is in the library).
Second, a TOFMS has the ability to carry out spectral deconvolution that mathematically separates spectral overlap for co-eluting analytes. This also plays a vital role in “purifying” a mass spectrum to achieve a better library match (Figure 4). Lastly, due to these factors, integrated software can automate the selection, search and identification of unknown peaks and develop a peak table. Despite the fact that manual review is still required to verify the outcomes of the automated peak find, there is a considerable reduction in the overall time needed to produce a list of identified peaks.
.jpg)
Figure 4. Side-by-side comparison of a GC-TOFMS and GCxGC-TOFMS analysis of the same pet food sample. (A) In the GC-TOFMS mass spectrum the flavoring compound 2-propionylthiazole had a library similarity of 660. Spectral deconvolution was not able to resolve (or identify) a co-eluting compound with m/z 136 in the peak true mass spectrum. (B) In the GCxGC-TOFMS contour plot the two co-eluting compounds were chromatographically resolved in the second dimension. The library similarity for 2-propionylthiazole was improved to 891 and the peak with m/z 136 was identified as 2-acetyl-3-methylpyrazine with a library similarity of 860 (not shown). Image courtesy of Joe Binkley, LECO Corporation.
Accelerate Discovery with Increased Peak Capacity
One of the most perfect, and most promoted, usages of GCxGC is the separation and identification of all of the individual components in a sample. Sample characterization can be essential to drug discovery, forensic investigations, and early disease diagnosis. Upon developing a GCxGC technique to enhance chromatographic resolution (i.e. True Peak Capacity Increase) [5, 15], a fingerprint of the sample can be acquired, which would be useful for source apportionment in environmental forensics. The indicator molecules (or biomarkers) are usually small peaks in a GC chromatogram that can be easily hidden in a 1D-GC analysis (Figure 5).
.jpg)
Figure 5. GCxGC-TOFMS contour plot of a tar ball sample and suspected crude oil source. Extracted ion chromatograms (XIC) are magnified to show the similarity between the two samples. The hopanes are molecular fossils that are resistant to weathering and are important biomarkers that aid in source apportionment. In a 1D-GC analysis the hopanes are more difficult to identify due to interferences with late eluting hydrocarbons.
In metabolomics, it has been observed that small molecules are sensitive indicators of disease. These biomarkers are vital for gaining insights into the disease with the aim of treatment and prevention. Compared to a GC-MS technique, a validated GCxGC-TOFMS technique found out 11 more statistically important biomarkers in human serum samples [10]. The improved resolution of the GCxGC analysis was highly significant for the discovery of additional biomarkers. Moreover, the extra sensitivity offered by the modulation process of the GCxGC system enabled the used of a 30:1 split injection rather than a splitless injection with the GC-MS technique. A split injection increases column lifetime (less non-volatile material deposited onto column) and ensures reproducibility.
Save Money with Economical Detectors
Increase in resolution through the detector or the gas chromatograph is necessary for the quantification of the minor components of a complex sample. Mass spectrometry is a robust detector with the ability to spectrally resolve and identify various co-eluting peaks. In a one-dimensional GC-MS analysis, huge differences in the concentration of co-eluting compounds, structural isomers, or multiple analytes eluting at the same instance can reduce the utility of the MS.
One method to increase the specificity of the MS is the use of high-resolution mass spectrometry (HRMS) or tandem mass spectrometry (MS/MS). Although both of these detectors are used in an analytical laboratory, they are very costly to buy and operate. The need for high-cost mass spectrometer detectors can be decreased by increasing the chromatographic resolution with GCxGC. The FID is a robust, economical, and simple-to-operate detector.
In total, 20 laboratories took part in an inter-calibration experiment using certified reference materials of Gulf of Mexico Crude Oil (SRM 2779). The laboratories were given the task of reporting the levels of different constituents of crude oil (for example, aromatics, saturated hydrocarbons, hopane and sterane biomarkers) in the NIST certified reference material [16].
Majority of the laboratories adopted GC-MS for the analysis; three laboratories adopted GC-MS/MS; and two laboratories adopted GCxGC-FID. In general, the reported values using GCxGC-FID were in good accordance with the NIST certified reference values.
The GCxGC-FID exhibited a performance analogous to GC-MS in the measurement of n-alkanes [17]. Only 8 of the 20 laboratories reported values for a list of various hopane and sterane biomarkers, one among which was the lab equipped with a GCxGC-FID. One more advantage of using an FID rather than mass spectrometry is the ease of quantification, specifically for hydrocarbons. An FID’s reliable detector response reduces the need for multiple internal standards to accomplish accurate quantitation.
Conclusion
An initial investment of resources is required for any advancement in technology. When it comes to investing in GCxGC, capital equipment purchases, staff training, and instrument operation costs are all important factors. Careful buyers might question whether the technology is applicable to a routine environment, or the time taken for it to realize a return on the investment.
The examples in this article provide insights into the applicability of GCxGC in a routine laboratory. Implementation of a GCxGC system can offer an immediate payoff in terms of saving time in sample preparation, instrumental analysis, and non-target data review. The potential for the analysis of a broad array of complex samples with simultaneous target and non-target detection is an appealing attribute that can be leveraged in a competitive industry. No longer is the comprehensive two-dimensional gas chromatography just a research hype; it is a futuristic separation technology.
References and Further Reading
- M. Golay, Theory of chromatography in open and coated tubular columns with round and rectangular cross-sections, in: D.H. Desty (Ed.) Gas Chromatography 1958, Butterworths Scientific Publications, 1958, pp. 36-55.
- R.D. Dandeneau, E.H. Zerenner, An investigation of glasses for capillary chromatography, Journal of High Resolution Chromatography, 2 (1979) 351-356.
- Z. Liu, J.B. Phillips, Comprehensive Two-Dimensional Gas Chromatography using an On-Column Thermal Modulator Interface, Journal of Chromatographic Science, 29 (1991) 227-231.
- LECO’s Pegasus 4D The Next Dimension in GC-TOFMS Analysis, in: Pegasus 4D, St. Joseph, MI, 2002.
- J. Cochran, Eight Tips for Easy GCxGC, in, The Analytical Scientist, 2014.
- LECO, Simply GCxGC, in, 2017.
- H.G.J. Mol, H. van der Kamp, G. van der Weg, M. van der Lee, A. Punt, T.C. de Rijk, Validation of Automated Library-Based Qualitative Screening of Pesticides by Comprehensive Two-Dimensional Gas Chromatography/Time-of-Flight Mass Spectrometry, Journal of AOAC International, 94 (2011) 1722-1740.
- A.M. Muscalu, E.J. Reiner, S.N. Liss, T. Chen, G. Ladwig, D. Morse, A routine accredited method for the analysis of polychlorinated biphenyls, organochlorine pesticides, chlorobenzenes and screening of other halogenated organics in soil, sediment and sludge by GCxGC-μECD, Analytical and Bioanalytical Chemistry, 401 (2011) 2403-2413.
- M. Menéndez-Carreño, H. Steenbergen, H.-G. Janssen, Development and validation of a comprehensive two-dimensional gas chromatography–mass spectrometry method for the analysis of phytosterol oxidation products in human plasma, Analytical and Bioanalytical Chemistry, 402 (2012) 2023-2032.
- J.H. Winnike, X. Wei, K.J. Knagge, S.D. Colman, S.G. Gregory, X. Zhang, Comparison of GC-MS and GCxGC-MS in the analysis of human serum samples for biomarker discovery, J Proteome Res, 14 (2015) 1810-1817.
- P. López, M. Tienstra, A. Lommen, H.G.J. Mol, Validation of an automated screening method for persistent organic contaminants in fats and oils by GCxGC-ToFMS, Food Chemistry, 211 (2016) 645-653.
- A.M. Muscalu, The Determination of Polychlorinated Biphenyl Congeners (PCBc), Organochlorines (OCs) and Chlorobenzenes (CBs) in Water Samples by GCxGC-μECD, in, Ministry of the Environment and Climate Change Laboratory Services Branch, Toronto, Ontario, Canada.
- A.M. Muscalu, The Determination of Polychlorinated Biphenyls (PCBs) in Biota Samples by GCxGC-μECD, in, Ministry of the Environment and Climate Change Laboratory Services Branch, Toronto, Ontario, Canada.
- A.M. Muscalu, The Determination of Polychlorinated Biphenyl Congeners (PCBc), Organochlorines (OCs) and Chlorobenzenes (CBs) in Solids by Two-Dimensional Gas Chromatography Micro-Electron Capture Detection (GCxGC-μECD), in, Ministry of the Environment and Climate Change Laboratory Services Branch, Toronto, Ontario, Canada, 2017, pp. 65.
- M.S. Klee, J. Cochran, M. Merrick, L.M. Blumberg, Evaluation of conditions of comprehensive two-dimensional gas chromatography that yield a near-theoretical maximum in peak capacity gain, Journal of Chromatography A, 1383 (2015) 151-159.
- J.A.A. Murray, L.C.C. Sander, S.A. Wise, C.M. Reddy, Gulf of Mexico Research Initiative 2014/2015 Hydrocarbon Intercalibration Experiment: Description and Results for SRM 2779 Gulf of Mexico Crude Oil and Candidate SRM 2777 Weathered Gulf of Mexico Crude Oil, in, NIST National Institute of Standards and Technology, 2016.
- C.M. Reddy, S.A. Wise, L.C. Sander, J.A. Murray, Summary of results from the 2014/2015 Hydrocarbon Intercalibration Experiment (HIE), in, 2015.
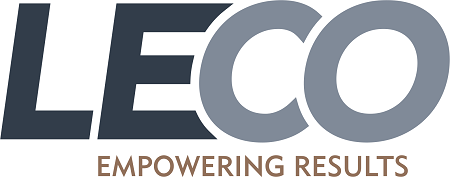
This information has been sourced, reviewed and adapted from materials provided by LECO Corporation, originally written by Michelle Misselwitz.
For more information on this source, please visit LECO Corporation.