The field of materials science has evolved significantly. Material design and development have progressed from traditional, expensive experimentation techniques to contemporary, data-driven AI-based algorithms.1
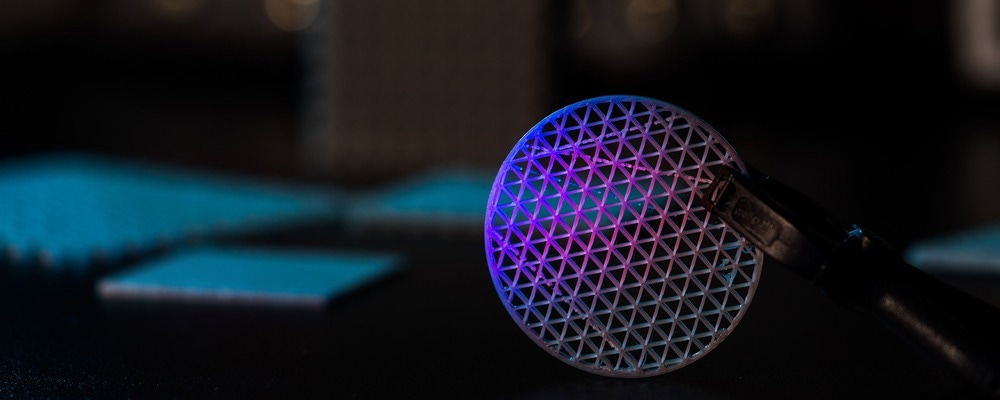
Image Credit: luchschenF/Shutterstock.com
The rapid development of materials through digital techniques has accelerated progress in various industries, particularly electric vehicle battery materials, renewable energy, construction, and aerospace. Among the various classes of materials, metamaterials stand out due to their remarkably unusual properties, such as electromagnetism, which are not typically found in conventional substances.2
Metamaterials: A Unique Artificially Engineered Material Type
Metamaterials are artificial materials comprised of an array of nanostructures, which serve as the building blocks, or "meta-atoms." Unlike natural materials, the properties of metamaterials are not determined solely by the intrinsic characteristics of their chemical constituents but rather by the precise arrangement of these meta-atoms.
The intricate interactions between incident electromagnetic (EM) waves and the meta-atoms endow metamaterials with remarkable light-field manipulation capabilities and other unique physical properties, some of which surpass those found in naturally occurring materials.3
Initially, the design of metamaterials was focused on optical and electromagnetic properties. However, it has now expanded to encompass mechanical (including both quasi-static and elasto-dynamic behavior), acoustic, biomedical, and thermal properties.4
This innovative class of functional materials is artificially engineered with unique micro- and nanoscale patterns or structures, enabling unprecedented interactions with light and other forms of energy. These composite materials are intentionally designed and fabricated to exploit their internal microstructures, efficiently achieving distinctive properties.
Their structure consists of multiple identical elements made of traditional materials, such as metals or non-conductive substances. These are often described as customizable artificial "atoms" and "molecules." The individual unit cell (or meta-atom) is typically much smaller than the waves interacting with the metamaterials.
The distribution between the individual structures is also relatively smaller than the wavelength of general EM waves. Engineers can tailor the shape, size, and lattice constant of these structures while manipulating the interactions between them. Strategically positioned "defects" can additionally be employed to enhance desired characteristics.5
Metamaterials: Applications Across Industries
Metamaterials find applications across various fields, including public safety, sensor identification, high-frequency battlefield communications, enhanced ultrasonic sensors, solar energy management for high-gain antennas, and remote aerospace applications.
In the military sector, scientists utilize metamaterials for several purposes, including the detection of explosive substances and the identification of contaminants and biological materials.6 Metamaterials also play a significant role in the development of compact and high-speed photonic devices.
Nano-scale metamaterials are employed to manipulate optical or acoustic signals, enhancing ultrasound resolution and material distortion. Metamaterials are also utilized in antennas to improve the efficiency of compact antenna systems. Metamaterial antennas leverage their exceptional bandwidth characteristics and unique structure to enhance antenna performance.6
Designing and Fabricating Metamaterials: An Overview
In the design phase of metamaterials, the primary objective is to engineer microscale architectures that can be manipulated to bestow specific macroscale properties on the final product. This design process typically involves the application of physical reasoning, analytical models, and computational techniques, collectively known as "rational design" approaches.7
The metamaterial design process is also becoming faster due to the emergence of rapid fabrication techniques, such as additive manufacturing (AM), which enables the construction of complex 3D structures through a layer-by-layer material deposition process.
While subtractive manufacturing predominates in the production of mechanical metamaterials, AM is an equally important cutting-edge technique. Two-photon polymerization (TPP) has been employed to manufacture structures with resolutions approaching 100 nm, resulting in microscale unit cell sizes.8
Selective laser sintering (SLS) has also been utilized in the fabrication of 3D metamaterials using plastic in 3D printing processes. Unlike previous 3D printing technologies, SLS involves sintering and fusing a preheated polymer powder with a laser to produce the printed metamaterial piece.9
Most of the proposed optical metamaterials with nanometer-sized features have been fabricated using conventional nano-patterning methods, such as Electron Beam Lithography (EBL) and Focused Ion Beam milling (FIB). However, these methods typically restrict the size of the fabricated metamaterials to very thin and, at most, micrometer-sized areas, significantly limiting the practical applications of metamaterials.
What Are the Main Challenges in Metamaterial Fabrication?
The fabrication of metamaterials has faced several limitations, including restricted pattern design, design inflexibility, material selection challenges, uncontrollability, and long-range disorder.10
One major barrier to the rapid commercialization of metamaterials is the operational challenges they present, as these substances operate at scales much smaller than the wavelength of the incident waves, such as light or microwaves.
Fabricating these functional materials with precise dimensions at the subwavelength level is exceptionally challenging, requiring extreme precision with tightly controlled reaction parameters and highly specialized equipment. These factors also increase the fabrication costs of metamaterials.
Many metamaterials also exhibit narrow bandwidths due to their resonant nature. Materials scientists are struggling with the design of wideband metamaterials that maintain their properties over a broader frequency range.
Artificial Intelligence and Tech Innovations: Transforming Metamaterials
The advancement and accessibility of computational techniques, including those leveraging artificial intelligence (AI), combined with the availability of cloud computing resources, have facilitated the enhanced exploration of the design space for metamaterials. These computational tools enable more comprehensive analyses and more sophisticated approaches to the rational design of metamaterials.7
Machine learning techniques have introduced new possibilities in the design of metamaterials. Firstly, they enable the solution of inverse design problems using deep learning and other AI tools. Secondly, generative models like generative adversarial networks (GANs) and variational auto-encoders (VAEs) can now assist in the rational design process by generating designs that match specific target properties.7,11
Multilayer perceptrons (MLPs) are a type of feedforward artificial neural network featuring fully connected neurons, nonlinear activation functions, and a structure consisting of at least three layers. MLPs have been used to predict various properties of phononic metamaterials. In the design of phononic metamaterials, an MLP can be trained using target properties as inputs and design variables as outputs.
Integrating MLPs with genetic algorithms has also facilitated the design of multifunctional elastic metasurfaces for precise control over wave refraction angles, yielding satisfactory results. MLPs can predict transmission coefficients and local phase shifts across different frequencies.11
Practical applications of mechanical metamaterials often involve solving inverse problems to identify microarchitectures that exhibit desired properties. However, the limited resolution of AM techniques necessitates tailoring these inverse solutions for specific specimen sizes.
Addressing the multi-objective inverse design challenges is vital for the real-world application of mechanical metamaterials. To overcome this size-agnostic inverse design problem, deep learning (DL) and deep generative models are employed within the random-network (RN) mechanical metamaterials framework.12
Recently, a modular approach called "Deep-DRAM" has been introduced to address size-agnostic inverse design challenges in mechanical metamaterials.12 Deep-DRAM combines four independent models, including two DL models, a deep generative model utilizing conditional variational autoencoders, and direct finite element (FE) simulations.
This integrated framework enables the identification of multiple solutions to the multi-objective inverse design problem, particularly focusing on random-network unit cells. Combining deep generative models with forward predictors has effectively generated mechanical metamaterials that meet various design criteria, including minimum peak stresses. This approach enhances the durability and endurance of these materials, making them suitable for practical applications in the real world.
In a recent development, the University of Exeter has secured significant funding to spearhead a groundbreaking collaboration to advance research into manipulating metamaterials in the fourth dimension: time. This initiative involves experts from Exeter’s Centre for Metamaterial Research and Innovation (CMRI), who will play a pivotal role in a newly formed consortium named Meta4D.
The consortium aims to explore the manipulation of waves, including light waves or acoustic waves, by utilizing tailored metamaterials that exhibit variations in time instead of space.13 This initiative underscores the scientific community’s belief in the critical role of metamaterials for future innovations.
Metamaterials are the subject of extensive research, and the integration of modern digital tools such as AI and data-driven models is driving the accelerated development of these substances, along with controlled manipulation of their properties. The future of metamaterials is promising, with expectations of significant advancements in the field in the coming years.
To learn more about the application of AI in materials science: The Intersection Between AI and 3D Printing
References and Further Reading
[1] Prateek, S., et. al. (2023). Data-driven materials science: application of ML for predicting band gap. Advances in Materials and Processing Technologies. doi.org/10.1080/2374068X.2023.2171666
[2] Kshetrimayum, R. (2005). A brief intro to metamaterials. IEEE Potentials. doi.org/10.1109/MP.2005.1368916
[3] Chen, J., et al. (2023). Metamaterials: from fundamental physics to intelligent design. Interdisciplinary Materials. doi.org/10.1002/idm2.12049
[4] Krushynska O. et al. (2023). Emerging topics in nanophononics and elastic, acoustic, and mechanical metamaterials: an overview. Nanophotonics. doi.org/10.1515/nanoph-2022-0671
[5] Nanowerk. (2023). Explaining metamaterials and metasurfaces – properties and applications. [Online] Nanowerk. Available at: https://www.nanowerk.com/what-are-metamaterials.php [Accessed: 12 March 2024]
[6] Valipour A., et al. (2022). Metamaterials and their applications: An overview. Proceedings of the Institution of Mechanical Engineers, Part L: Journal of Materials: Design and Applications. Available at: https://doi.org/10.1177/1464420721995858
[7] Zadpoor, A. et. al. (2023). Design, material, function, and fabrication of metamaterials. APL Materials. doi.org/10.1063/5.0144454
[8] Jin, H., et al. (2024). Mechanical metamaterials fabricated from self-assembly: A perspective. Journal of Applied Mechanics. doi.org/10.1115/1.4064144
[9] Alex-Amor, A., et al. (2022). 3-D Metamaterials: Trends on Applied Designs, Computational Methods and Fabrication Techniques. Electronics. doi.org/10.3390/electronics11030410
[10] Yoon, G., et al. (2016). Challenges in fabrication towards realization of practical metamaterials. Microelectronic Engineering. doi.org/10.1016/j.mee.2016.05.005
[11] Liu, X., et al. (2024). Machine learning models in phononic metamaterials. Current Opinion in Solid State and Materials Science. doi.org/10.1016/j.cossms.2023.101133
[12] Pahlavani, H., et al. (2024). Deep Learning for Size‐Agnostic Inverse Design of Random‐Network 3D Printed Mechanical Metamaterials. Advanced Materials.doi.org/10.1002/adma.202303481
[13] Sandes, D. (2024). Research to build next generation of metamaterials receives multi-million pound funding boost. [Online] University of Exeter. Available at: https://news.exeter.ac.uk/top-stories/research-to-build-next-generation-of-metamaterials-receives-multi-million-pound-funding-boost/ [Accessed on 16 March 2024]
Disclaimer: The views expressed here are those of the author expressed in their private capacity and do not necessarily represent the views of AZoM.com Limited T/A AZoNetwork the owner and operator of this website. This disclaimer forms part of the Terms and conditions of use of this website.